Advertisement

A Review of Design Consideration, Challenges and Technologies Used in 5G Antennas
- Published: 18 March 2023
- Volume 129 , pages 1585–1621, ( 2023 )
Cite this article
- Tapan Nahar 1 , 2 &
- Sanyog Rawat ORCID: orcid.org/0000-0002-1001-3070 3
697 Accesses
2 Citations
Explore all metrics
The telecommunications industry is one of the fastest growing sector. Technological upgradation is required every year to provide better service quality, coverage and capacity and providing more new features. Fifth generation (5G) is the most recent mobile generation that will offer high-speed internet and data services, minimal-latency services, reliable connections, ultra-high resolution multimedia services, and access to billions of devices which leads to possible the applications such as fully automated production industries, driverless autonomous car, tele-surgery and many more. 5G mobile networks will provide better service quality, coverage and capacity. Larger bandwidth is necessary to provide high data rate services. Due to a lack of frequency spectrum, the currently utilized microwave band is incapable of meeting the 5G objective. So, while the mm-Wave spectrum has a huge number of frequency bands available, high link loss and environmental absorptions are key limits that may be solved by constructing a wide band antenna with high gain, high efficiency, and a steerable narrow radiating beam. The most crucial component of a wireless communication system is the antenna, which transmits and receives radio waves. Microstrip antennas are popular for a variety of applications because to their small size, light weight, low profile, and simple fabrication, but they have some limitations, including low radiation efficiency, low gain, restricted bandwidth, and others. There are several problems and major issues that have yet to be resolved. To attain high performance, low cost, and planar layout, upcoming 5G technology necessitates certain alterations in standard antenna design methodologies. The objective of this study is to address 5G antenna design challenges and barriers, as well as to identify research gaps in this area. It will also cover possible antenna technologies used in antenna design, a review of some recently created antenna solutions, and performance comparisons. To attain the higher performance necessary for 5G and to solve design difficulties, many approaches such as massive MIMO, electromagnetic band gap, substrate integrated waveguide, metamaterials, metasurface, artificial magnetic conductor, dielectric superstrate, butler matrix, and others have been used in 5G antennas. Every approach has advantages and disadvantages, which are explained in this paper. By appropriately combining these strategies, one may obtain the requisite antenna performance for 5G. A review of current developments in 5G antennas based on these approaches is reviewed, along with their merits and limits.
This is a preview of subscription content, log in via an institution to check access.
Access this article
Price excludes VAT (USA) Tax calculation will be finalised during checkout.
Instant access to the full article PDF.
Rent this article via DeepDyve
Institutional subscriptions
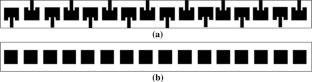
Similar content being viewed by others
Fundamentals of Antenna Design, Technologies and Applications
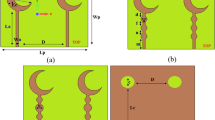
Design and Analysis of a Compact MIMO Antenna for 5G mmWave N257, N260, and N262 Band Applications
Nizar Sghaier, Anouar Belkadi, … Ali Gharsallah
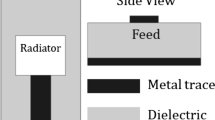
Implementational Aspects of Various Feeding Techniques for mmWave 5G Antennas
G. S. Karthikeya, M. Idrees Magray, … Shiban K. Koul
Data Availability
Not applicable
Code availability
(software application or custom code): Not Applicable.
A White Paper on Enabling 5 G in India. (2019). TRAI .
Rappaport, T. S., Sun, S., Mayzus, R., Zhao, H., Azar, Y., Wang, K., & Gutierrez, F. (2013). Millimeter wave mobile communications for 5G cellular: It will work! IEEE Access, 1 , 335–349. https://doi.org/10.1109/ACCESS.2013.2260813
Article Google Scholar
Habaebi, M. H., Janat, M., & Rafiqul, I. M. (2018). Beam steering antenna array for 5G telecommunication systems applications. Progress In Electromagnetics Research M, 67 , 197–207. https://doi.org/10.2528/PIERM17091802
O’Hara, J. F., Ekin, S., Choi, W., & Song, I. (2019). A Perspective on terahertz next-generation wireless communications. Technologies, 7 (2), 43. https://doi.org/10.3390/technologies7020043
Hong, W., Baek, K. H., Lee, Y., Kim, Y., & Ko, S. T. (2014). Study and prototyping of practically large-scale mmWave antenna systems for 5G cellular devices. IEEE Communications Magazine, 52 (9), 63–69. https://doi.org/10.1109/MCOM.2014.6894454
Kumar, S., Dixit, A. S., Malekar, R. R., Raut, H. D., & Shevada, L. K. (2020). Fifth generation antennas: A comprehensive review of design and performance enhancement techniques. IEEE Access, 8 , 163568–163593. https://doi.org/10.1109/ACCESS.2020.3020952
Uchendu, I., & Kelly, J. (2016). Survey of beam steering techniques available for millimeter wave applications. Progress In Electromagnetics Research B, 68 , 35–54. https://doi.org/10.2528/PIERB16030703
Larsson, E. G., Edfors, O., Tufvesson, F., & Marzetta, T. L. (2014). Massive MIMO for next generation wireless systems. IEEE Communications Magazine, 52 (2), 186–195. https://doi.org/10.1109/MCOM.2014.6736761
Sazegar, M., Zheng, Y., Kohler, C., Maune, H., Nikfalazar, M., Binder, J. R., & Jakoby, R. (2012). Beam steering transmitarray using tunable frequency selective surface with integrated ferroelectric varactors. IEEE Transactions on Antennas and Propagation, 60 (12), 5690–5699. https://doi.org/10.1109/TAP.2012.2213057
Askari, G., & Kamarei, M. (2017). Frequency and time domain design, analysis and implementation of a multi-gbps uwb wilkinson power divider for 5g new spectrum and CAR applications. Progress In Electromagnetics Research B, 77 , 103–116. https://doi.org/10.2528/PIERB17042204
Abdo, Y., Chaharmir, M. R., Shaker, J., & Antar, Y. M. M. (2013). Detailed study of millimeter wave EBG guide: Broadbanding techniques, modal structure, and crosstalk behavior. Progress In Electromagnetics Research B, 50 , 141–156. https://doi.org/10.2528/PIERB13022010
Roh, W., Seol, J.-Y., Park, J., Lee, B., Lee, J., Kim, Y., & Aryanfar, F. (2014). Millimeter-wave beamforming as an enabling technology for 5G cellular communications: theoretical feasibility and prototype results. IEEE Communications Magazine, 52 (2), 106–113. https://doi.org/10.1109/MCOM.2014.6736750
Gandhi, O. P., & Riazi, A. (1986). Absorption of millimeter waves by human beings and its biological implications. IEEE Transactions on Microwave Theory and Techniques, 34 (2), 228–235. https://doi.org/10.1109/TMTT.1986.1133316
Zhadobov, M., Chahat, N., Sauleau, R., Le Quement, C., & Le Drean, Y. (2011). Millimeter-wave interactions with the human body: State of knowledge and recent advances. International Journal of Microwave and Wireless Technologies, 3 (2), 237–247. https://doi.org/10.1017/S1759078711000122
Zhou, H., & Aryanfar, F. (2013). Millimeter-wave open ended SIW antenna with wide beam coverage. In 2013 IEEE Antennas and Propagation Society International Symposium (APSURSI) (pp. 658–659). https://doi.org/10.1109/APS.2013.6710989
Mohajer, M., Faraji-Dana, M., & Safavi-Naeini, S. (2014). Effects of resonance-based phase shifters on Ka-band phased array antenna performance for satellite communications. Progress In Electromagnetics Research B, 60 , 259–274. https://doi.org/10.2528/PIERB14060906
Khalily, M., Tafazolli, R., Xiao, P., & Kishk, A. A. (2018). Broadband mm-wave microstrip array antenna with improved radiation characteristics for different 5G applications. IEEE Transactions on Antennas and Propagation, 66 (9), 4641–4647. https://doi.org/10.1109/TAP.2018.2845451
Mao, C. X., Khalily, M., Xiao, P., Brown, T. W. C., & Gao, S. (2019). Planar sub-millimeter-wave array antenna with enhanced gain and reduced sidelobes for 5G broadcast applications. IEEE Transactions on Antennas and Propagation, 67 (1), 160–168. https://doi.org/10.1109/TAP.2018.2874796
Khalily, M., Tafazolli, R., Rahman, T. A., & Kamarudin, M. R. (2016). Design of phased arrays of series-fed patch antennas with reduced number of the controllers for 28-GHz mm-wave applications. IEEE Antennas and Wireless Propagation Letters, 15 , 1305–1308. https://doi.org/10.1109/LAWP.2015.2505781
Khalily, M., Tafazolli, R., Rahman, T. A., & Kamarudin, M. (2016). Design of phased arrays of series-fed patch antennas with reduced number of the controllers for 28-GHz mm-wave applications. IEEE Antennas and Wireless Propagation Letters, 15 , 1305–1308.
Roy, P., Vishwakarma, R. K., Jain, A., & Singh, R. (2016). Multiband millimeter wave antenna array for 5G communication. In 2016 International Conference on Emerging Trends in Electrical Electronics Sustainable Energy Systems (ICETEESES) (pp. 102–105). https://doi.org/10.1109/ICETEESES.2016.7581361
Huang, H.-C. (2018). Overview of antenna designs and considerations in 5G cellular phones. In 2018 International Workshop on Antenna Technology (iWAT) (pp. 1–4). https://doi.org/10.1109/IWAT.2018.8379253
Sanyog Rawat Tapan Nahar. (2021). Survey of 5G antenna for industrial automation applications. In Recent trends in Sciences, Engineering and Social Sciences (pp. 19–33). Manipal University Jaipur.
Nahar, T., & Rawat, S. (2021). Survey of various bandwidth enhancement techniques used for 5G antennas. International Journal of Microwave and Wireless Technologies . https://doi.org/10.1017/S1759078720001804
Liu, Q., Mkongwa, K. G., & Zhang, C. (2021). Performance issues in wireless body area networks for the healthcare application: A survey and future prospects. SN Applied Sciences, 3 (2), 155. https://doi.org/10.1007/s42452-020-04058-2
Ghanmi, A., Varsier, N., Hadjem, A., Conil, E., Picon, O., & Wiart, J. (2013). Study of the influence of the laterality of mobile phone use on the SAR induced in two head models. Comptes Rendus Physique, 14 (5), 418–424. https://doi.org/10.1016/j.crhy.2013.02.007
Samineni, P., Khan, T., & De, A. (2017). Modeling of electromagnetic band gap structures: A review. International Journal of RF and Microwave Computer-Aided Engineering, 27 (2), e21055. https://doi.org/10.1002/mmce.21055
Babu, N., Ansari, A. Q., Gangwar, D., Kanaujia, B., Kumar, S., & Gupta, S. (2022). Dual-band circularly-polarized EBG-based antenna for Wi-MAX/WLAN/ISM band applications. Wireless Personal Communications . https://doi.org/10.1007/s11277-022-09951-0
Padhi, S. K., & Bialkowski, M. E. (2003). A microstrip yagi antenna using EBG structure. Radio Science . https://doi.org/10.1029/2002RS002697
ITU-R. (2015). Studies on frequency-related matters for International Mobile Telecommunications identification including possible additional allocations to the mobile services on a primary basis in portion (s) of the frequency range between 24. 25 and 86 GHz for the. World Radiocommunication Conference, 238 , 25–27.
Google Scholar
Alheety, A., Islam, M., Rashid, A., Fadil, A., & Arabian, F. (2020). Performance evaluation of wireless data traffic in mm wave massive MIMO communication. Indonesian Journal of Electrical Engineering and Computer Science . https://doi.org/10.11591/ijeecs.v20.i3.pp1342-1350
Xu, H.-X., Tang, S., Sun, C., Li, L., Liu, H., Yang, X., & Sun, Y. (2018). High-efficiency broadband polarization-independent superscatterer using conformal metasurfaces. Photonic Research, 6 (8), 782–788. https://doi.org/10.1364/PRJ.6.000782
Senthilkumar, S., Surendar, U., Christina, X. S., & William, J. (2022). A compact phased array antenna for 5G MIMO applications. Wireless Personal Communications . https://doi.org/10.1007/s11277-022-10037-0
Verma, A., Arya, R. K., & Nallanthighal, R. (2022). Wireless personal communications metasurface superstrate beam steering antenna with AMC for 5G/WiMAX/WLAN applications. Wireless Personal Communications . https://doi.org/10.1007/s11277-022-09993-4
Dey, S., Kiran, N. S., & Dey, S. (2020). SIW butler matrix driven beam scanning array for millimeter wave 5G communication. In 2020 IEEE Asia-Pacific Microwave Conference (APMC) (pp. 709–711). https://doi.org/10.1109/APMC47863.2020.9331670
Wang, Z., Dai, X., & Sun, W. (2020). Tri-beam slot antenna array based on substrate integrated waveguide (SIW) technology. International Journal of Microwave and Wireless Technologies, 12 (3), 246–251. https://doi.org/10.1017/S1759078719001260
Wan, W., Wang, Q., & Ye, T. (2019). Research of low-profile high performance new AMC antenna for 5G mm-wave AiP application. In 2019 20th International Conference on Electronic Packaging Technology (ICEPT) (pp. 1–4). https://doi.org/10.1109/ICEPT47577.2019.245133
Liu, F., Zhang, Z., Lu, X., Gan, W., Yang, J., & Yang, S. (2020). Design of a Simple Antenna Based on AMC Reflector for Dual Ultra Broadband Applications. In 2020 Asia Conference on Computers and Communications (ACCC) (pp. 25–28). https://doi.org/10.1109/ACCC51160.2020.9347929
Ibrahim, A. A., & Ali, W. A. E. (2021). High gain, wideband and low mutual coupling AMC-based millimeter wave MIMO antenna for 5G NR networks. AEU - International Journal of Electronics and Communications, 142 , 153990. https://doi.org/10.1016/j.aeue.2021.153990
Dilli, R. (2022). Hybrid beamforming in 5G nr networks using multi user massive MIMO at FR2 frequency bands. Wireless Personal Communications . Springer US. https://doi.org/10.1007/s11277-022-09952-z
Gharbi, I., Barrak, R., Menif, M., Ribero, J. M., Diallo, A., & Ragad, H. (2019). High gain patch antenna array using dielectric superstrate for the 5G applications. In 2019 IEEE 19th Mediterranean Microwave Symposium (MMS) (pp. 1–4). https://doi.org/10.1109/MMS48040.2019.9157289
Haraz, O. M., Elboushi, A., Alshebeili, S. A., & Sebak, A.-R. (2014). Dense dielectric patch array antenna with improved radiation characteristics using EBG ground structure and dielectric superstrate for future 5G cellular networks. IEEE Access, 2 , 909–913. https://doi.org/10.1109/ACCESS.2014.2352679
Dilli, R. (2020). Analysis of 5G Wireless Systems in FR1 and FR2 Frequency Bands. In 2020 2nd International Conference on Innovative Mechanisms for Industry Applications (ICIMIA) (pp. 767–772). https://doi.org/10.1109/ICIMIA48430.2020.9074973
5G spectrums and the worldwide gap: Where do regulators stand? (2020). Telecom Review . Retrieved December 9, 2020, from https://www.telecomreview.com/index.php/special-editions/2020/3781-5g-mena-2020
Studies, C. for E. P. (2016). Global 5G spectrum update, (June). Retrieved from https://www.ceps.eu/sites/default/files/imagefield_thumbs/Luigi Ardito CEPS 26102016 BRUXELLES.pdf
Tikhomirov, A., Omelyanchuk, E., & Semenova, A. (2018). Recommended 5G frequency bands evaluation. In 2018 Systems of Signals Generating and Processing in the Field of on Board Communications (pp. 1–5). https://doi.org/10.1109/SOSG.2018.8350639
Wang, Y., Li, J., Huang, L., Jing, Y., Georgakopoulos, A., & Demestichas, P. (2014). 5G mobile: Spectrum broadening to higher-frequency bands to support high data rates. IEEE Vehicular Technology Magazine, 9 (3), 39–46. https://doi.org/10.1109/MVT.2014.2333694
Shimodaira, H., Tran, G. K., Sakaguchi, K., & Araki, K. (2015). Investigation on millimeter-wave spectrum for 5G. In 2015 IEEE Conference on Standards for Communications and Networking (CSCN) (pp. 143–148). https://doi.org/10.1109/CSCN.2015.7390435
Diawuo, H. A., & Jung, Y.-B. (2018). Broadband proximity-coupled microstrip planar antenna array for 5G cellular applications. IEEE Antennas and Wireless Propagation Letters, 17 (7), 1286–1290. https://doi.org/10.1109/LAWP.2018.2842242
Stanley, M., Huang, Y., Wang, H., Zhou, H., Alieldin, A., & Joseph, S. (2018). A Capacitive coupled patch antenna array with high gain and wide coverage for 5G smartphone applications. IEEE Access, 6 , 41942–41954. https://doi.org/10.1109/ACCESS.2018.2860795
Kuo, F.-Y., & Hwang, R.-B. (2014). High-isolation X-band marine radar antenna design. IEEE Transactions on Antennas and Propagation, 62 (5), 2331–2337. https://doi.org/10.1109/TAP.2014.2307296
Agarwal, D. S. (2021). Concurrent 60/94 GHz SIR based planar antenna for 5G/MM-wave imaging applications. Wireless Personal Communications, 121 , 1–11. https://doi.org/10.1007/s11277-021-08691-x
Deb, P., & De, D. (2022). Sustainable spectrum allocation strategy for 5G mobile network. Wireless Personal Communications, 125 , 1–24. https://doi.org/10.1007/s11277-022-09738-3
Ojaroudiparchin, N., Shen, M., Zhang, S., & Pedersen, G. F. (2016). A switchable 3-D-coverage-phased array antenna package for 5G mobile terminals. IEEE Antennas and Wireless Propagation Letters, 15 , 1747–1750. https://doi.org/10.1109/LAWP.2016.2532607
Asma, K., Wakrim, L., Saida, I., & Hassani, M. (2022). Beam-steerable ultra-wide-band miniaturized elliptical phased array antenna using inverted-L-shaped modified inset feed and defected ground structure for 5G smartphones millimeter-wave applications. Wireless Personal Communications . https://doi.org/10.1007/s11277-022-09737-4
Andrews, J. G., Buzzi, S., Choi, W., Hanly, S. V., Lozano, A., Soong, A. C. K., & Zhang, J. C. (2014). What will 5G be? IEEE Journal on Selected Areas in Communications, 32 (6), 1065–1082. https://doi.org/10.1109/JSAC.2014.2328098
Yassin, M. E., Mohamed, H. A., Abdallah, E. A. F., & El-Hennawy, H. S. (2019). Single-fed 4G/5G multiband 2.4/5.5/28 GHz antenna. IET Microwaves, Antennas and Propagation, 13 (3), 286–290. https://doi.org/10.1049/iet-map.2018.5122
Yu, B., Yang, K., Sim, C.-Y.-D., & Yang, G. (2018). A novel 28 GHz beam steering array for 5G mobile device with metallic casing application. IEEE Transactions on Antennas and Propagation, 66 (1), 462–466. https://doi.org/10.1109/TAP.2017.2772084
Li, G., Zhai, H., Ma, Z., Liang, C., Yu, R., & Liu, S. (2014). Isolation-improved dual-band MIMO antenna array for LTE/WiMAX mobile terminals. IEEE Antennas and Wireless Propagation Letters, 13 , 1128–1131. https://doi.org/10.1109/LAWP.2014.2330065
Mao, C.-X., Gao, S., & Wang, Y. (2017). Broadband high-gain beam-scanning antenna array for millimeter-wave applications. IEEE Transactions on Antennas and Propagation, 65 (9), 4864–4868. https://doi.org/10.1109/TAP.2017.2724640
Viikari, V., Luomaniemi, R., Ala-Laurinaho, J., Kurvinen, J., Kähkönen, H., Lehtovuori, A., & Leino, M. (2019). 5G Antenna Challenges and Opportunities. In 2019 16th International Symposium on Wireless Communication Systems (ISWCS) (pp. 330–334). https://doi.org/10.1109/ISWCS.2019.8877314
Frølund-Pedersen, G. (2013). Mobile phone antenna performance 14.
Krogerus, J., Toivanen, J., Icheln, C., & Vainikainen, P. (2007). effect of the human body on total radiated power and the 3-D radiation pattern of mobile handsets. IEEE Transactions on Instrumentation and Measurement, 56 (6), 2375–2385. https://doi.org/10.1109/TIM.2007.903591
Hannula, J.-M., Saarinen, T., Holopainen, J., & Viikari, V. (2017). Frequency reconfigurable multiband handset antenna based on a multichannel transceiver. IEEE Transactions on Antennas and Propagation, 65 (9), 4452–4460. https://doi.org/10.1109/TAP.2017.2725384
Kurvinen, J., Kähkönen, H., Lehtovuori, A., Ala-Laurinaho, J., & Viikari, V. (2019). Co-designed mm-wave and LTE handset antennas. IEEE Transactions on Antennas and Propagation, 67 (3), 1545–1553. https://doi.org/10.1109/TAP.2018.2888823
Wu, Z., Wu, B., Su, Z., & Zhang, X. (2018). Development challenges for 5G base station antennas. In 2018 International Workshop on Antenna Technology (iWAT) (pp. 1–3). https://doi.org/10.1109/IWAT.2018.8379163
Li, L., Niu, X., Chai, Y., Chen, L., Zhang, T., Cheng, D., & You, X. (2016). The path to 5G: mm wave aspects. Journal of Communications and Information Networks, 1 (2), 1–18. https://doi.org/10.1007/bf03391553
Nguyen, N. L., & Vu, V. Y. (2019). Gain enhancement for MIMO antenna using metamaterial structure. International Journal of Microwave and Wireless Technologies, 11 (8), 851–862. https://doi.org/10.1017/S175907871900059X
Article MathSciNet Google Scholar
Wu, J., Cheng, Y. J., & Fan, Y. (2016). Millimeter-wave wideband high-efficiency circularly polarized planar array antenna. IEEE Transactions on Antennas and Propagation, 64 (2), 535–542. https://doi.org/10.1109/TAP.2015.2506726
Article MathSciNet MATH Google Scholar
Akbari, M., Gupta, S., Farahani, M., Sebak, A. R., & Denidni, T. A. (2017). Analytic study on CP enhancement of millimeter wave DR and patch subarray antennas. International Journal of RF and Microwave Computer-Aided Engineering, 27 (1), e21053. https://doi.org/10.1002/mmce.21053
MANTASH, M., Kakhki, M. B., & DENIDNI, T. A. (2018). Millimeter-Wave Circularly Polarized Vivaldi Antenna Using Simple Single Layer 2D FSS Polarizer. In 2018 18th International Symposium on Antenna Technology and Applied Electromagnetics (ANTEM) (pp. 1–2). https://doi.org/10.1109/ANTEM.2018.8572921
Tong, K.-F., & Wong, T.-P. (2007). Circularly polarized U-slot antenna. IEEE Transactions on Antennas and Propagation, 55 (8), 2382–2385. https://doi.org/10.1109/TAP.2007.901930
Chen, A., Zhang, Y., Chen, Z., & Cao, S. (2010). A ka-band high-gain circularly polarized microstrip antenna array. Antennas and Wireless Propagation Letters, IEEE, 9 , 1115–1118. https://doi.org/10.1109/LAWP.2010.2093866
Mantash, M., & Denidni, T. A. (2019). CP antenna array with switching-beam capability using electromagnetic periodic structures for 5G applications. IEEE Access, 7 , 26192–26199. https://doi.org/10.1109/ACCESS.2019.2901440
Mittra, R. (2018). Some Challenges in Millimeter Wave Antenna Designs for 5G. In 2018 International Symposium on Antennas and Propagation (ISAP) (pp. 1–2).
Kamran Shereen, M., Khattak, M. I., & Witjaksono, G. (2019). A brief review of frequency, radiation pattern, polarization, and compound reconfigurable antennas for 5G applications. Journal of Computational Electronics, 18 (3), 1065–1102. https://doi.org/10.1007/s10825-019-01336-0
Ehyaie, D., & Mortazawi, A. (2011). A 24-GHz modular transmit phased array. IEEE Transactions on Microwave Theory and Techniques, 59 (6), 1665–1672. https://doi.org/10.1109/TMTT.2011.2140122
Garcia-Marin, E., Masa-Campos, J. L., & Sanchez-Olivares, P. (2019). Planar array topologies for 5G communications in ku band [Wireless Corner]. IEEE Antennas and Propagation Magazine, 61 (2), 112–133. https://doi.org/10.1109/MAP.2019.2895633
Ershadi, E., Keshtkar, A., Abdelrahman, A., Xin, H., & Ershadi, E. (2017). Wideband high gain antenna subarray for 5G application. Progress In Electromagnetics Research C . https://doi.org/10.2528/PIERC17061301
Yin, J., Wu, Q., Yu, C., Wang, H., & Hong, W. (2019). Broadband symmetrical E-shaped patch antenna with multimode resonance for 5G millimeter-wave applications. IEEE Transactions on Antennas and Propagation, 67 (7), 4474–4483. https://doi.org/10.1109/TAP.2019.2911266
Sodré, A. C., da Costa, I. F., dos Santos, R. A., Filgueiras, H. R. D., & Spadoti, D. H. (2018). Waveguide-based antenna arrays for 5G networks. International Journal of Antennas and Propagation, 2018 , 5472045. https://doi.org/10.1155/2018/5472045
Nahar, T., & Rawat, S. (n.d.). Efficiency enhancement techniques of microwave and millimeter-wave antennas for 5G communication: A survey. Transactions on Emerging Telecommunications Technologies . Doi: https://doi.org/10.1002/ett.4530
Nahar, T., & Rawat, S. (2020). Low Cost Planar Millimeter Wave Antenna Array for 5G Mobile Applications. In 2020 2nd International Conference on Advances in Computing, Communication Control and Networking (ICACCCN) (pp. 621–626). https://doi.org/10.1109/ICACCCN51052.2020.9362828
Marzouk, H. M., Ahmed, M. I., & Shaalan, A. A. (2019). A Novel Dual-band 28/38 GHz Slotted Microstip MIMO Antenna for 5G Mobile Applications. In 2019 IEEE International Symposium on Antennas and Propagation and USNC-URSI Radio Science Meeting (pp. 607–608). https://doi.org/10.1109/APUSNCURSINRSM.2019.8888799
Malhat, H., Elhenawy, A., Zainud-Deen, S., & Al-Shalaby, N. (2022). Planar reconfigurable plasma leaky-wave antenna with electronic beam-scanning for MIMO applications. Wireless Personal Communications . https://doi.org/10.1007/s11277-022-09972-9
Mantash, M., & Tarot, A.-C. (2016). On the bandwidth and geometry of dual-band AMC structures. In 2016 10th European Conference on Antennas and Propagation (EuCAP) (pp. 1–4). https://doi.org/10.1109/EuCAP.2016.7481589
Peng, L., Ruan, C. L., & Xiong, J. (2012). Compact EBG for multi-band applications. IEEE Transactions on Antennas and Propagation, 60 (9), 4440–4444. https://doi.org/10.1109/TAP.2012.2207036
Chen, H. N., Song, J.-M., & Park, J.-D. (2019). A compact circularly polarized mimo dielectric resonator antenna over electromagnetic band-gap surface for 5G applications. IEEE Access, 7 , 140889–140898. https://doi.org/10.1109/ACCESS.2019.2943880
Shen, X., Liu, Y., Zhao, L., Huang, G.-L., Shi, X., & Huang, Q. (2019). A miniaturized microstrip antenna array at 5G millimeter-wave band. IEEE Antennas and Wireless Propagation Letters, 18 (8), 1671–1675. https://doi.org/10.1109/LAWP.2019.2927460
Dadgarpour, A., Zarghooni, B., Virdee, B. S., & Denidni, T. A. (2015). Enhancement of tilted beam in elevation plane for planar end-fire antennas using artificial dielectric medium. IEEE Transactions on Antennas and Propagation, 63 (10), 4540–4545. https://doi.org/10.1109/TAP.2015.2456937
Modak, S., Surender, D., Shome, P., & Khan, T. (2022). Switchable/Tunable Band-notched characteristics in UWB and UWB-MIMO antennas: A comprehensive review. Wireless Personal Communications . https://doi.org/10.1007/s11277-022-10036-1
Jeyakumar, D., Malar, E., Srinitha, S., Muthuchidambaranathan, P., & Ramesh, A. (2022). Hybrid beamforming in large-scale antenna array for 5G indoor communication network deployments. Wireless Personal Communications . https://doi.org/10.1007/s11277-022-09808-6
Ben Mabrouk, I., Al-Hasan, M., Nedil, M., Denidni, T. A., & Sebak, A.-R. (2020). A novel design of radiation pattern-reconfigurable antenna system for millimeter-wave 5G applications. IEEE Transactions on Antennas and Propagation, 68 (4), 2585–2592. https://doi.org/10.1109/TAP.2019.2952607
Li, M., Luk, K.-M., Ge, L., & Zhang, K. (2016). Miniaturization of magnetoelectric dipole antenna by using metamaterial loading. IEEE Transactions on Antennas and Propagation, 64 (11), 4914–4918. https://doi.org/10.1109/TAP.2016.2599176
Chaimool, S., Rakluea, C., & Akkaraekthalin, P. (2013). Mu-near-zero metasurface for microstrip-fed slot antennas. Applied Physics A, 112 (3), 669–675. https://doi.org/10.1007/s00339-013-7703-6
Chaimool, S., Chung, K., & Akkaraekthalin, P. (2010). Simultaneous gain and bandwidths enhancement of a single-feed circularly polarized microstrip patch antenna using a metama-Terial reflective surface. Progress in Electromagnetics Research B, 22 , 23–37. https://doi.org/10.2528/PIERB10031901
Xu, H.-X., Tang, S., Wang, G.-M., Cai, T., Huang, W., He, Q., & Zhou, L. (2016). Multifunctional microstrip array combining a linear polarizer and focusing metasurface. IEEE Transactions on Antennas and Propagation, 64 (8), 3676–3682. https://doi.org/10.1109/TAP.2016.2565742
Cai, T., Wang, G., Zhang, X.-F., Wang, Y., Zong, B., & Xu, H. (2015). Compact microstrip antenna with enhanced bandwidth by loading magneto-electro-dielectric planar waveguided metamaterials. IEEE Transactions on Antennas and Propagation, 63 , 2306–2311.
Zhu, S., Liu, H., & Wen, P. (2019). A new method for achieving miniaturization and gain enhancement of vivaldi antenna array based on anisotropic metasurface. IEEE Transactions on Antennas and Propagation, 67 (3), 1952–1956. https://doi.org/10.1109/TAP.2019.2891220
Liu, W., Chen, Z. N., & Qing, X. (2015). Metamaterial-based low-profile broadband aperture-coupled grid-slotted patch antenna. IEEE Transactions on Antennas and Propagation, 63 (7), 3325–3329. https://doi.org/10.1109/TAP.2015.2429741
Liu, W., Chen, Z. N., & Qing, X. (2014). 60-GHz thin broadband high-gain LTCC metamaterial-mushroom antenna array. IEEE Transactions on Antennas and Propagation, 62 (9), 4592–4601. https://doi.org/10.1109/TAP.2014.2333052
Article MATH Google Scholar
Dey, S., & Dey, S. (2020). EBG Superstrate Loaded Circularly Polarized Fabry-Perot Cavity Antenna at Sub-6 GHz for Satellite and 5G Cellular Communications. In 2020 International Symposium on Antennas & Propagation (APSYM) (pp. 101–104). https://doi.org/10.1109/APSYM50265.2020.9350725
Denidni, T. A., & Libar, T. E. (2003). Wide band four-port butler matrix for switched multibeam antenna arrays. In 14th IEEE Proceedings on Personal, Indoor and Mobile Radio Communications, 2003. PIMRC 2003. (Vol. 3, pp. 2461–2464). https://doi.org/10.1109/PIMRC.2003.1259161
Nedil, M., Denidni, T., Djaiz, A., & Habib, A. (2008). A new ultra-wideband beamforming for wireless communications in underground mines. Progress in Electromagnetics Research M, 4 , 1–21. https://doi.org/10.2528/PIERM08070207
Download references
No funding source.
Author information
Authors and affiliations.
Department of Electronics and Communication Engineering, Manipal University Jaipur, Jaipur, India
Tapan Nahar
Department of Information and Communication Technology, Marwadi University, Rajkot, India
Department of Electronics and Communication Engineering, Central University of Rajasthan, Ajmer, India
Sanyog Rawat
You can also search for this author in PubMed Google Scholar
Corresponding author
Correspondence to Sanyog Rawat .
Ethics declarations
Conflicts of interest.
There is no conflicts of interest.
Additional information
Publisher's note.
Springer Nature remains neutral with regard to jurisdictional claims in published maps and institutional affiliations.
Rights and permissions
Springer Nature or its licensor (e.g. a society or other partner) holds exclusive rights to this article under a publishing agreement with the author(s) or other rightsholder(s); author self-archiving of the accepted manuscript version of this article is solely governed by the terms of such publishing agreement and applicable law.
Reprints and permissions
About this article
Nahar, T., Rawat, S. A Review of Design Consideration, Challenges and Technologies Used in 5G Antennas. Wireless Pers Commun 129 , 1585–1621 (2023). https://doi.org/10.1007/s11277-023-10193-x
Download citation
Accepted : 08 February 2023
Published : 18 March 2023
Issue Date : April 2023
DOI : https://doi.org/10.1007/s11277-023-10193-x
Share this article
Anyone you share the following link with will be able to read this content:
Sorry, a shareable link is not currently available for this article.
Provided by the Springer Nature SharedIt content-sharing initiative
- Fifth Generation (5G)
- Microstrip antenna
- Mobile communication
- Beamforming
- Find a journal
- Publish with us
- Track your research
antenna design Recently Published Documents
Total documents.
- Latest Documents
- Most Cited Documents
- Contributed Authors
- Related Sources
- Related Keywords
Investigation on Performance of Microstrip Patch Antenna for a Practical Wireless Local Area Network (WLAN) Application
Abstract: The performance of a microstrip patch antenna for a practical wireless local area network application is investigated in this research. This design is built around the transmission line concept. The antenna design substrate is FR4 (lossy) with a dielectric constant (Er) of 4.3 dielectric material, and the ground and patch materials are copper (annealed). The substrate is 71.62mm in width and 55.47mm in length. The height of the dielectric material is 1.6mm, which is the normal size for FR4 material. The conducting patch element has a width of 35.81mm and a length of 27.73mm for a resonance frequency of 2.573 GHz. A simulation with CST studio suite was used to optimise the antenna design. Keywords: Microstrio patch antenna, CST suite, WLAN application, Transmission line, Antenna design
Performance Improvement of Aperture Coupled MSA through Si Micromachining
In recent times rectangular patch antenna design has become the most innovative and popular subject due to its advantages, such as being lightweight, conformal, ease to fabricate, low cost and small size. In this paper design of aperture coupled microstrip patch antenna (MSA) on high index semiconductor material coupled with micromachining technique for performance enhancement is discussed. The performance in terms of return loss bandwidth, gain, cross-polarization and antenna efficiency is compared with standard aperture coupled antenna. Micromachining underneath of the patch helps in to reduce the effective dielectric constant, which is desirable for the radiation characteristics of the patch antenna. Improvement 36 percent and 18 percent in return loss bandwidth and gain respectively achieved using micromachined aperture coupled feed patch, which is due to the reduction in losses, suppression of surface waves and substrate modes. In this article along with design, fabrication aspects on Si substrate using MEMS process also discussed. Presented antenna design is proposed antenna can be useful in smart antenna arrays suitable in satellite, radar communication applications. Two topologies at X-band are fabricated and comparison between aperture coupled and micromachined aperture coupled are presented. Index Terms—Microstrip Patch Antenna, Aperture Coupled, Micromachining, High Resistivity Silicon
Isolation enhancement of metamaterial structure MIMO antenna for WiMAX/WLAN/ITU band applications
Abstract The μ-negative metamaterial (MNG) two-element MIMO antenna design was proposed in this article for WiMAX (2.5–2.8 GHz), WLAN (3.2–5.9 GHz), and ITU band (8.15−8.25 GHz) applications. The first design of the MIMO antenna operates at 2.7 and 4.9 GHz frequencies. In order to reduce the mutual coupling, a defective ground structure is used. For further isolation improvement, an MNG unit cell is placed in between the two radiating elements at a distance of 10 mm. The designed antenna elements have better than −23 dB coupling isolation between the two radiating elements. Moreover, with MNG an additional frequency of 8.2 GHz is obtained, which is useful for ITU band applications. The proposed antenna bandwidth is expanded by 19% in the lower operational band, 20% in the second operational band, and 32% in the higher frequency band with the MNG unit cell. From the analysis, the proposed antenna is suitable for WiMAX/WLAN/ITU band applications because of its low enveloped correlation coefficient, and highest directive gain and low mutual coupling between the radiating components. The proposed antenna was simulated, fabricated, and measured with the help of the Schwarz ZVL vector network analyzer and anechoic chamber. Both measured and simulated results are highly accurate and highly recommended for WiMAX/WLAN/ITU bands.
5G/B5G Internet of Things MIMO Antenna Design
The current and future wireless communication systems, WiFi, fourth generation (4G), fifth generation (5G), Beyond5G, and sixth generation (6G), are mixtures of many frequency spectrums. Thus, multi-functional common or shared aperture antenna modules, which operate at multiband frequency spectrums, are very desirable. This paper presents a multiple-input and multiple-output (MIMO) antenna design for the 5G/B5G Internet of Things (IoT). The proposed MIMO antenna is designed to operate at multiple bands, i.e., at 3.5 GHz, 3.6 GHz, and 3.7 GHz microwave Sub-6 GHz and 28 GHz mm-wave bands, by employing a single radiating aperture, which is based on a tapered slot antenna. As a proof of concept, multiple tapered slots are placed on the corner of the proposed prototype. With this configuration, multiple directive beams pointing in different directions have been achieved at both bands, which in turn provide uncorrelated channels in MIMO communication. A 3.5 dBi realized gain at 3.6 GHz and an 8 dBi realized gain at 28 GHz are achieved, showing that the proposed design is a suitable candidate for multiple wireless communication standards at Sub-6 GHz and mm-wave bands. The final MIMO structure is printed using PCB technology with an overall size of 120 × 60 × 10 mm3, which matches the dimensions of a modern mobile phone.
Recent Advances in Antenna Design for 5G Heterogeneous Networks
Fifth-generation will support significantly faster mobile broadband speeds, low latency, and reliable communications, as well as enabling the full potential of the Internet of Things (IoT) [...]
Improved Carbon Fiber Reinforced Plastic Structural Antenna Design for near-HF Remote Sensing Applications
A planar low-profile meander antenna design for wireless terminal achieving low rf interference and high isolation in multi-antenna systems, multi frequency antenna design based on binary coding, dual-band rfid tag antenna design for uhf band applications with high read range performance, low profile uhf antenna design for low earth-observation cubesats, export citation format, share document.
Thank you for visiting nature.com. You are using a browser version with limited support for CSS. To obtain the best experience, we recommend you use a more up to date browser (or turn off compatibility mode in Internet Explorer). In the meantime, to ensure continued support, we are displaying the site without styles and JavaScript.
- View all journals
- My Account Login
- Explore content
- About the journal
- Publish with us
- Sign up for alerts
- Open access
- Published: 23 November 2023
Selection of metallic liquid in sub-6 GHz antenna design for 6G networks
- Sasmita Dash 1 ,
- Constantinos Psomas 1 &
- Ioannis Krikidis 1
Scientific Reports volume 13 , Article number: 20551 ( 2023 ) Cite this article
1440 Accesses
Metrics details
- Electrical and electronic engineering
- Electronic devices
- Electronics, photonics and device physics
The rapid evolution of wireless communication systems toward 6G demands efficient antennas with the features of adaptability and versatility. Liquid antennas have gained significant research interest due to their unique features in realizing small, flexible, transparent, and reconfigurable antennas for promising applications in future wireless systems. In this paper, in order to find a suitable metallic liquid for effective antennas, we design and compare the performance of metallic liquid antennas using Mercury, gallium indium alloy (EGaIn), and Graphene metallic liquid in the sub-6 GHz frequency. The antenna is realized by the metallic liquid in a poly methyl methacrylate microfluidic channel over a liquid crystal polymer substrate at 5.6 GHz frequency. The performance of these metallic liquid antennas is analyzed by their electromagnetic and radiation performance. The Graphene-based metallic liquid antenna shows better electromagnetic performance in comparison to Mercury and EGaIn metallic liquid antennas.
Similar content being viewed by others
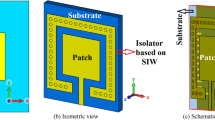
High-isolation antenna array using SIW and realized with a graphene layer for sub-terahertz wireless applications
Mohammad Alibakhshikenari, Bal S. Virdee, … Ernesto Limiti
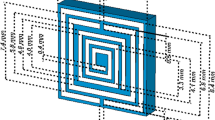
Dual square split ring enclosed spiral shaped hybrid metamaterial resonator with size miniaturisation for microwave wireless applications
Air Mohammad Siddiky, Mohammad Rashed Iqbal Faruque, … K. S. Al-Mugren
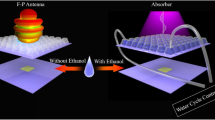
Reconfigurable integrated structures with functions of Fabry–Perot antenna and wideband liquid absorber for radar system stealth
Yukun Zou, Xiangkun Kong, … Yongjiu Zhao
Introduction
Communication technologies are undergoing a revolutionary shift due to the rapid progress of communication applications. Cellular mobile communication systems have gone through five generations of development. 5G is the latest generation of wireless networks. 5G has already been introduced in some regions of the world and officially became commercially available in 2019, employing sub-6 GHz and mm-wave frequency bands 1 . For a point-to-point link, the mm-wave communication systems generally suffer more loss than sub-6 GHz systems 2 , 3 . Sub-6 GHz is substantially reasonable for carriers to implement because the range below 6 GHz is longer and penetrates objects in a better way.
In today’s wireless communications system, adaptability and diversified functionality have become the most appealing features of any communications device. The antenna has great importance for the communication system because the design of the air interface largely depends on the design of the antenna. The rapid evolution of wireless communication systems towards 6G demands efficient antennas. As conventional antennas are mostly made up of conductive metals on rigid substrates, they offer remarkable performance but lack mechanical flexibility. Furthermore, bending or stretching these antennas beyond certain limits causes irreversible structural deformation and even destruction 4 . The rigid nature of metallic antennas limits their use in applications that require flexibility. On the other hand, the flowing property and having no deformation limits of metallic liquid make them an ideal alternative for flexible antenna applications. Without sacrificing their electrical properties, liquid metals in microfluidic channels develop extremely flexible and mechanically stable antennas 5 . Modern fabrication techniques for antennas, such as 3D printing, injecting, or spraying metallic liquid onto rigid or flexible substrates, are made possible by the fluidic property of metallic liquids. In contrast to conventional techniques like high-frequency switching, liquid antennas can be easily achieved reconfigurability through electrochemically controlled capillary action or micro pumping. Liquid antennas take advantage of the fluid’s mechanical properties. The presence of metallic liquid in fluidic channels enables the formation of the shape of a fluidic channel due to its low viscosity 6 . Liquid antennas on flexible substrates can bend, fold, stretch, and twist. Therefore, they can stand up to all kinds of mechanical deformation. Due to their high degree of reversibility, they are capable of regaining their original form 7 .
Liquid metal antennas were introduced in the 1990s to make flexible and reconfigurable antennas 8 , 9 . A comprehensive literature review reveals that Mercury, and alloys of gallium and tin, are utilized in the design of metallic liquid antennas 5 , 10 , 11 , 12 . Mercury is the first used metallic liquid for antenna design. The fluidic and conductive property of Mercury enables the design of reconfigurable metallic liquid antenna. However, Mercury is toxic in nature and expensive. These facts boost the use of other metallic liquid materials gallium-based alloys 5 , 11 , 12 . Alloys based on gallium have remarkable electrical and thermal conductivity, non-toxicity and low viscosity 13 , 14 . In addition to these properties, gallium indium alloy (EGaIn) has mechanical stability and significantly prevents evaporation 14 . These features of EGaIn help in the improvement of antenna performance without having any effect on the conductivity and efficiency of the antenna. The most recent material that has been reported for antenna design is Graphene. Graphene is emerging as a promising candidate in several applications due to its electrical, thermal, and optical properties. It has excellent material properties for electronic applications. Graphene antennas perform much better than conventional copper metal antennas 15 , 16 , 17 . However, the Graphene metallic liquid for antenna design is not yet found in the literature. Finding the best metallic liquid material for metallic antenna design is vital for the antenna community. This information is pertinent from the perspective of the antenna designer.
In this paper, we design and numerically analyze metallic liquid antennas by using Mercury, EGaIn, and Graphene liquid. We compare the electromagnetic performance of these metallic liquid antennas. A metallic liquid antenna with a working frequency of 5.6 GHz is designed as the candidate antenna to verify the suitability of a specific metallic liquid. In particular, the main contributions of the paper are summarized as follows:
We consider three antennas using metallic liquids of Mercury (Hg), an alloy of gallium and indium (EGaIn), and Graphene. The metallic liquid is injected in a poly methyl methacrylate (PMMA) microfluidic channel and the antenna is placed over a metallic grounded liquid crystal polymer (LCP) substrate.
We investigate the numerical analysis of three different metallic liquid antennas and compare the radiation performance of these antennas in terms of gain, efficiency, and bandwidth.
We design metallic liquid based antennas for sub-6 GHz systems by using the electromagnetic (EM) simulator Ansys HFSS.
Materials for metallic liquid antennas
Due to their inherent flexibility, liquid materials are a good substitute for stiff or solid conductors in flexible electronics 18 . The fluidic properties of metallic liquids enable a wide range of metallic liquid antennas 5 , 8 , 9 , 10 , 11 , 12 . The fluid nature of liquid materials provides additional degrees of freedom to achieve better reconfigurability, even when the radiative elements are created on rigid substrates.
The basis for creating effective liquid antennas is liquid materials. The antenna design and performance mainly result from the properties of the liquid. The liquid materials that are readily available for antenna design can generally be divided into three categories: metallic, non-metallic, and partially metallic liquids. This work primarily focuses only on metallic liquids. Electrical conductivity is typically high in metallic liquids, which is suitable for use as radiating elements in antennas.
Due to their solid-like oxide coating on the surface, metallic liquids give elastomeric antennas with mechanical stability and flexibility. The high conductivity of metallic liquid makes it ideal for antenna applications. The use of metallic liquids as radiative elements enables the design of a considerably highly reconfigurable and flexible antenna than solid conductors like copper. The metallic liquid antenna is ideal for antenna applications due to its high flexibility and deformability, as well as its high conductivity.
The first metallic liquid at room temperature is Mercury (Hg). Alternative metallic liquid materials are mostly in the form of alloys of conductive nanoparticles. Gallium-based alloys exhibit low viscosities, high electrical and thermal conductivities, and non-toxicity. These metallic liquids typically have conductivities of the order of \(10^6\) S/m, which is high enough to achieve high radiation efficiency when employed as antenna materials. In this work, Graphene, a new class of new and noble metallic liquid, is introduced in addition to Mercury and gallium-based alloys.
Mercury (Hg)
Mercury is found in its liquid phase at room temperature; Mercury does conduct electricity because it has a metallic property and excellent volume expansion properties with the rise in temperature. The electrons of Mercury are free to move to conduct the flow of electric flux on the surface of the matter. A sufficient amount of electric flux penetrates through the Mercury per unit cross-section area. Mercury can carry electricity when it’s in liquid or molten form. The electrical conductivity of liquid Mercury is due to the transfer of electrons that takes place in the liquid or molten state.
Mercury has a melting point of \(-38.87\,^\circ\) C, a density \(@ 25\,^\circ\) C of 13.55 g/cm \(^{3}\) , and an electrical conductivity of \(1.0 \times 10^{6}\) S/m 19 . In addition, due to its high stiction and low oxidation properties, it is a suitable material for the development of a liquid antenna 10 . Mercury, on the other hand, is very toxic and has to be handled very carefully. Therefore, it has limited usage in antenna design.
Gallium Indium alloy (EGaIn)
Gallium-based alloys exhibit remarkable electrical and thermal conductivities, low viscosity, and non-toxicity. These materials have a melting point that’s either lower than or near room temperature 13 . One of the most commonly used alloys based on gallium is the EGaIn alloy, which is composed of 75% gallium (Ga) and 25% indium (In). EGaIn has an electrical conductivity of \(3.4\times 10^{6}\) S/m and a density of 6.25 g/cm \(^{3}\) at \(25\,^\circ\) C. Furthermore, the melting point of EGaIn is close to room temperature, with a melting point of \(16\,^\circ\) C 13 , 14 . The resistivity of EGaIn is \(~29.4 \times 10^{-6}\) \(\Omega \,\) cm 14 . When the liquid alloy is exposed to air, it reacts with oxygen to create a thin oxide layer on the surface, which enhances mechanical stability and surface tension, and significantly inhibits evaporation. These benefits enhance EGaIn performance in metallic liquid antennas without having any effect on the conductivity and efficiency of antennas. The most significant feature of EGaIn metallic liquid is non-toxicity. The unique feature of EGaIn helps in designing reconfigurable and flexible antennas 5 , 11 , 12 .
Graphene liquid
A carbon-based new metallic liquid material is the liquid form of Graphene. Graphene liquid can be employed for the design of metallic liquid antennas. Because of the high mobility of electrons in the hexagonally arranged carbon atoms of Graphene, it has an electrical conductivity of \(\sim 50 \times 10^{6}\) S/m 20 . It is directly dependent on the electron mobility in the matter. The resistivity of Graphene remains between \(1.19 \times 10^{-6}\) ohm cm and \(1.87 \times 10^{-6}\) ohm cm. It was found that the electrical resistivity of Graphene is an order of magnitude lower than that of the indium-gallium alloy. Graphene liquid is conductive enough to be used as antenna material with high radiation efficiency. Graphene has no known melting point. The reason for this aspect is that it does not melt upon heating. Instead, it undergoes sublimation, which is the transformation of a solid into a gas without ever turning into a liquid. Sublimation of Graphene occurs at temperatures of approximately 3600 K.
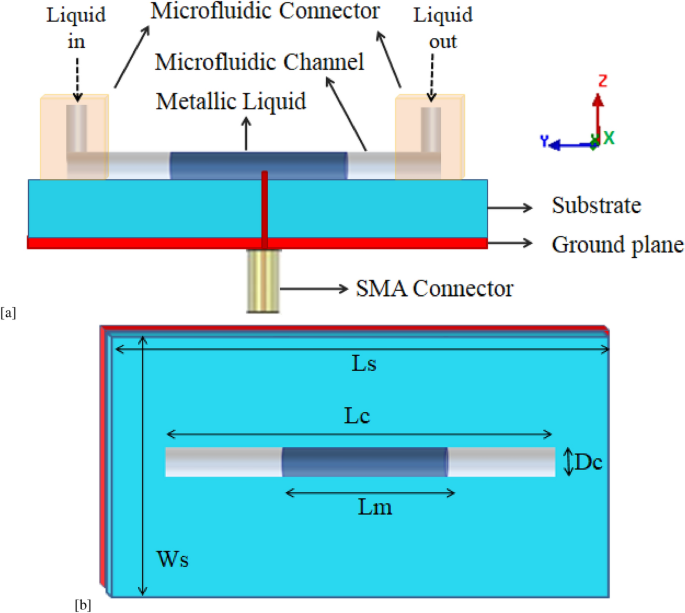
Schematic of the proposed metallic liquid antenna ( a ) Cross-sectional view and ( b ) 3D view.
Graphene was first isolated and characterized in 2004 21 . The superior properties of Graphene, including high electrical conductivity, high mechanical strength, adsorption capability, thermal resistance, etc., have led to a great deal of current research interest and a wide range of practical applications. Pristine Graphene has remarkable physical properties, including high electronic mobility ( \(\sim 250,000~ \hbox {cm}^{2}/\hbox {V}\,\hbox {s}\) ), excellent optical transparency (up to \(\sim 97.7\%\) ), and high electrical and thermal conductivity (over 3000 W/m K). In addition, Graphene with no defects or impurities, has great strength, rigidity, and elasticity. It has a mechanical strength of \(\sim 130~ \hbox {GPa}\) and an elasticity of \(\sim 1.0 ~\hbox {Tpa}\) . Graphene is \(100\%\) pure and stable. Liquid Graphene is safe to use in industry and environmentally friendly. Currently, liquid-based Graphene is found in various applications 22 . The utilization of Graphene inks for flexible electronic applications has been the subject of extensive research in recent years 23 . For the first time, we employ a Graphene-conductive liquid for antenna designs in this work. Table 1 presents the material properties of metallic liquids for antennas.
We design, numerically analyze, and compare the electromagnetic performance of three different metallic liquid antennas made up of Mercury, EGaIn and Graphene metallic liquid in the Sub-6 GHz frequency in order to find a suitable metallic liquid for effective Sub-6 GHz antennas. A metallic liquid in a microfluidic channel is considered as the candidate antenna for comparison of the radiation performance for these three metallic liquids Mercury, EGaIn, and Graphene. These three antennas are realized by injecting a fixed volume ( \(\approx\) 1 ml) of metallic liquid into a PMMA microfluidic channel of length 35 mm over a metallic grounded LCP substrate of dimension \((50 \times 30 \times 3) ~\hbox {mm}^{3}\) . For the proposed antenna structure, platinum metal is considered as a ground plane. Figure 1 a, b illustrate the 3D and cross-sectional view of the proposed metallic liquid antennas. The dimensions of the antennas are optimized for 5.6 GHz operational frequency. Geometrical antenna dimensions for this frequency of antenna are presented in Table 2 . Ansys HFSS (FEM-based Electromagnetic Solver) is used to simulate the antennas. These antennas are excited by using the center-fed single probe method. The ground plane (bottom layer) of the antennas is usually a sheet of metal that is electrically connected to the outside conductor of an SMA connector. From its bottom center, the feeding probe inserts into the metallic liquid and is connected electrically to the internal conductor of the SMA.
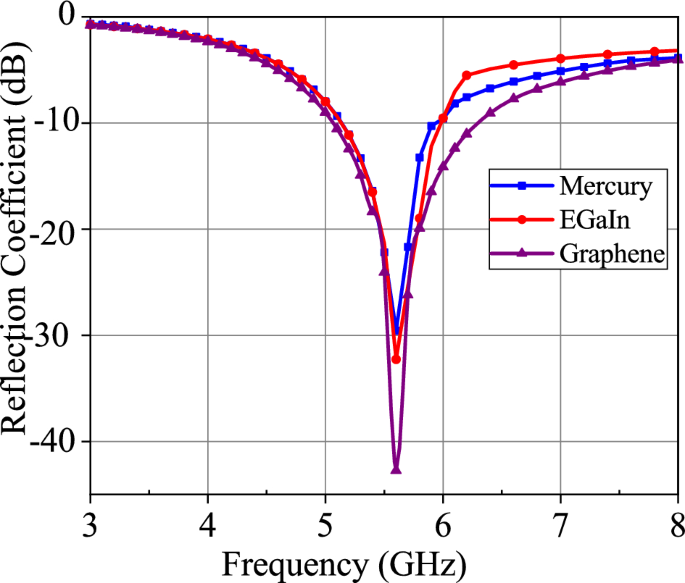
Reflection coefficient of Mercury, EGaIn and Graphene metallic liquid antennas.
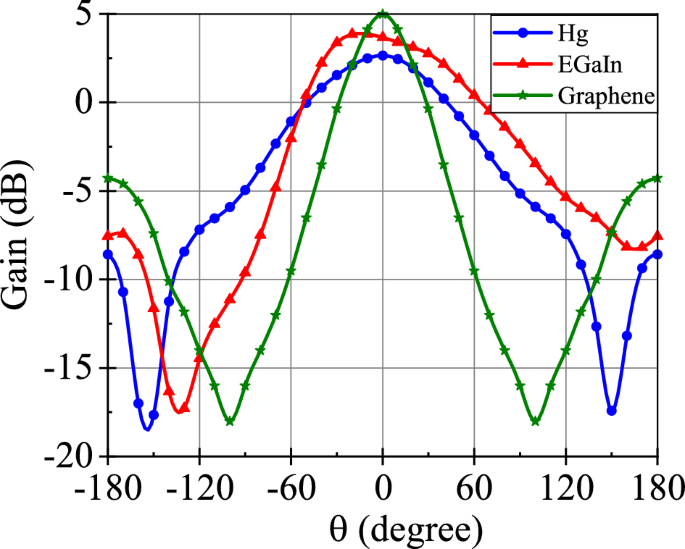
Radiation pattern of Mercury, EGaIn, and Graphene metallic liquid antennas at 5.6 GHz.
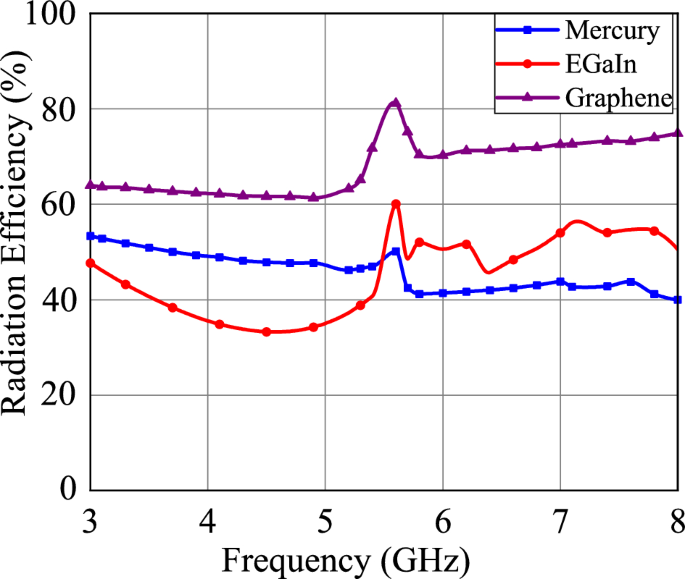
Radiation efficiency of Mercury, EGaIn, and Graphene metallic liquid antennas.
The reflection coefficients of Mercury, EGaIn, and Graphene metallic liquid antennas are shown in Fig. 2 . It can be noticed that these three antennas resonate at the same frequency 5.6 GHz, whereas the reflection coefficients of these metallic liquid antennas are different, such as − 29.5 dB, − 32 dB, and − 42 dB for Mercury, EGaIn, and Graphene metallic liquid antennas, respectively. The Graphene metallic liquid antennas have a low reflection coefficient and wider 10-dB impedance bandwidth in comparison to conventional Mercury and EGaIn metallic liquid antennas.
The radiation patterns of these three antennas are shown in Fig. 3 . Although the radiation patterns of the three antennas are almost similar, the difference in gain can be marked in Fig. 3 . At 5.6 GHz frequency, Mercury, EGaIn, and Graphene metallic liquid antennas have gain of 2.6 dBi, 3.6 dBi, and 5.0 dBi, respectively. The radiation efficiency of antennas is illustrated in Fig. 4 . The radiation efficiency of Graphene, EGaIn and Mercury metallic liquid antennas at 5.6 GHz are 81%, 60% and 50%, respectively. We can notice in Figs. 3 and 4 that the Graphene metallic liquid antenna has high gain and high radiation efficiency in comparison to Mercury and EGaIn metallic liquid antennas at operational frequency 5.6 GHz. The performance of these three metallic liquid antennas is summarized in Table 3 . Owing to superior conductivity and fluidic properties, Graphene metallic liquid antenna performs better than Mercury and EGaIn liquid antennas. EGaIn liquid antennas perform better than Mercury liquid antennas.
Graphene is particularly best in terms of loss when used as an antenna and its performance is influenced by the loss characteristics of each material. The material’s conductivity influences the losses in the antenna. The less conductive the metal, the more loss takes place. From Table 1 , it can be marked that Graphene has more conductivity than EGaIn and mercury. Therefore, Graphene metallic liquid antenna has a lower loss than EGaIn and mercury metallic liquid antenna.
The loss characteristics of each material in the operational frequency band further can be noticed in Fig. 2 . This illustrates the loss of Mercury, EGaIn, and Graphene metallic liquid antennas. At an operational frequency 5.6 GHz, Graphene metallic liquid antennas have a low loss in comparison to Mercury and EGaIn metallic liquid antennas. The loss characteristic can also be marked from the radiation efficiency of the antenna in Fig. 4 . Graphene metallic liquid antenna has high radiation efficiency in comparison to Mercury and EGaIn metallic liquid antennas at operational frequency 5.6 GHz. An antenna achieves high radiation efficiency if it has low loss. In the case of antenna with low radiation efficiency, more internal losses within the antenna takes place. Therefore, Graphene metallic liquid antenna has low loss compared to EGaIn and Mercury metallic liquid antenna.
The rapid growth of customer demands has necessitated the development of technologies and network capacity due to the major evolution of wireless technology from 1G to 6G. The sub-6GHz spectrum is used for optimal coverage in suburban and rural areas, whereas the mm-wave band is used in dense urban areas. The mm-wave networks are not feasible in rural and suburban areas due to their lack of range. In spite of the potential of multi-Gbps rates, several technical challenges exist in the mm-wave communication system 24 . The scope of mm-wave is likely to remain limited and will only be widely deployed in urban areas. With the growth of wireless generations and technologies, there has also been significant technological advancement in antenna design to meet the ever-growing requirements of customers 25 . The rapid growth of wireless communication systems necessitates the utilization of high-performance antennas in order to increase coverage and reduce the complexity of the system 26 , 27 , 28 , 29 . Henceforth, the 5G and 6G antennas should be efficient in terms of bandwidth, gain/directivity, efficiency, polarization, etc.
Unlike traditional conductors such as copper, metallic liquids have a flow property and lack of elasticity limit. This makes them ideal for applications that require mechanically flexible antennas. In general, conventional antennas are made with conductive metals like copper, which makes antennas very efficient but not flexible. By their nature, fluidic materials do not have any deformation limitations, making them the ideal candidates for flexible antenna applications. The liquid antenna has gained research interest due to its flexibility and reconfigurability feature. As far as metallic liquid antennas are concerned, the available metallic liquids are Mercury, EGaIn, and Graphene. For metallic liquid antenna design, Mercury is the first used metallic liquid and Graphene is the latest metallic liquid. The metallic liquid EGaIn, an alloy of gallium and tin metallic liquid also widely used for metallic liquid antenna design. The performance of metallic liquid antennas mainly depends on their fluidic and conductivity properties. In the sub-6 GHz spectrum, Graphene metallic liquid antenna with working frequency of 5.6 GHz performs better than EGaIn and Mercury metallic liquid antenna in terms of gain, bandwidth, reflection coefficient, and radiation efficiency.
Due to their inherent flexibility, liquid materials are a good substitute for stiff or solid conductors in flexible electronics. In addition to flexibility, another advantage of a metallic liquid antenna in a microfluidic channel is its reconfigurability. The fluid nature of liquid materials provides additional degrees of freedom to achieve better reconfigurability. Frequency reconfiguration and beam reconfiguration in metallic liquid antennas can be achieved by controlling the volume of liquid and the movement of liquid inside the microfluidic channel in different locations.
While offering unique advantages, metallic liquid antennas can indeed face challenges related to oxidation and residue formation when applied to microfluidic channels. This oxidation can create an oxide skin on the surface of the metallic liquid, which may affect the electrical properties of the antenna and potentially lead to the degradation of the antenna’s performance. Proper encapsulation or passivation techniques can be used to mitigate this issue. Treating the metallic liquid surface with a passivating material such as PMMA helps to prevent or reduce oxidation. Furthermore, metallic liquids can leave residues when they come into contact with certain materials. In microfluidic channels, these residues may lead to clogging or contamination of the channel, affecting the device’s performance. Properly selecting the materials used in the microfluidic channel helps mitigate residue formation. PMMA materials for the microfluidic channel are less susceptible to interaction with the metallic liquid and reduce the formation of residues and potential contamination.
The effects of oxidation and residues on liquid metal antennas in microfluidic channels vary depending on the specific liquid metal used, such as Mercury, EGaIn, and Graphene. Mercury is highly susceptible to oxidation. Oxidation can create an insulating layer on the surface of the metallic liquid, significantly reducing its electrical conductivity. This leads to a loss of antenna performance. Mercury leaves residues on the microfluidic channel walls over time. These residues can accumulate, leading to contamination and potential clogging of the channel. EGaIn can be generally preferred over mercury due to its reduced susceptibility to oxidation and the lower extent of residue formation. It has a less severe impact on electrical conductivity compared to mercury. EGaIn provides better antenna performance in terms of gain and radiation efficiency and reduces the risk of contamination and clogging. Graphene metallic liquids offer advantages in terms of oxidation mitigation and reduced residue formation. This helps in maintaining the electrical conductivity of the Graphene metallic liquid and antenna performance. Therefore, Graphene liquid metals provide better antenna performance in terms of reflection coefficient, gain and radiation efficiency compared to Mercury and EGaIn metallic liquid.
This article presents a comparative analysis of Mercury, EGaIn, and Graphene metallic liquid material for sub-6 GHz antenna design in order to find a metallic liquid for designing efficient antennas in this frequency regime. The electromagnetic performance of a metallic liquid antenna made up of Mercury, EGaIn, and Graphene metallic liquid with 5.6 GHz resonant frequency is simulated and numerically analyzed in an EM simulator. The performance of these three antennas at the same working frequency is quite different because of their fluidic and conductivity properties. The antenna made up of Graphene liquid performs better than the antenna made up of Mercury and EGaIn metallic liquid in terms of gain, bandwidth, reflection coefficient, and radiation efficiency. The overall conclusion is the best suitability of Graphene metallic liquid for the design of metallic liquid antennas operating for sub-6 GHz systems.
The Ansys HFSS, a finite element method (FEM)-based electromagnetic (EM) solver, is used to validate the proposed designed antenna 30 . Three different metallic liquid antennas of Mercury, EGaIn, and Graphene with a resonant frequency of 5.6 GHz are simulated. Three metallic liquid antennas are realized by injecting a fixed volume ( \(\approx\) 1 ml) of metallic liquid into a PMMA microfluidic channel of length 35 mm over a metallic grounded LCP substrate of dimension \((50 \times 30 \times 3) ~\hbox {mm}^{3}\) . The platinum metal is used as a ground plane for the proposed antenna structure. The volume of metallic liquid and dimensions of the antenna structures are optimized for 5.6 GHz operational frequency. In the EM simulation, Mercury ( \(\epsilon _{r}\) =1, tan \(\delta\) = 0.001, \(\sigma\) = \(1.0 \times 10^{6}\) S/m) and EGaIn ( \(\epsilon _{r}\) = 3.3, tan \(\delta\) = 0, \(\sigma\) = \(3.4 \times 10^{6}\) S/m) at operational frequency 5.6 GHz are used 30 . However, to model Graphene-based liquid, it is essential to model the conductive liquid with the surface conductivity \(\sigma _s\) of Graphene in the operational frequency 5.6 GHz according to Kubo formalism 31 . Therefore, Graphene liquid in the EM simulator is modelled as conductive liquid with surface conductivity \(\sigma _s\) .
where j is the imaginary unit, \(K_B\) is the Boltzmann’s constant, e is the electronic charge, T is the temperature, \(\omega\) is the angular frequency, \(\hbar\) is the reduced Planck’s constant, \(\tau\) is the relaxation time and \(\mu _c\) is the chemical potential.
The feeding mechanism of the proposed metallic liquid antenna can be illustrated with the help of Fig. 1 . These antennas are excited by using the center-fed single probe method. Antennas with metallic liquid radiating elements that have narrow cross sections (such as a dipole) are excited at the middle of the antenna structure. The feed structure of a metallic liquid antenna in a microfluidic channel typically involves connecting the external conductor of the SMA adapter to the ground plane. The feeding probe is inserted into the metallic liquid from its bottom center and it is electrically connected to the inner conductor of the SMA, as depicted in Fig. 1 . To ensure maximum radiation from the antenna, it is essential to achieve impedance matching. The antenna impedance matching behavior is shown in Fig. 2 . It can be seen that three antennas antenna possess a well-matched resonant frequency at 5.6 GHz.
The fabrication feasibility of the proposed metallic liquid antenna can be explained with the help of Fig. 1 a. Antennas with metallic liquid radiating elements that have narrow cross sections (such as a dipole) can be fabricated by simply injecting the metallic liquid into microfluidic channel. In this work, three antennas using Mercury, EGaIn, and Graphene metallic liquid can be realized by injecting metallic liquid into a PMMA microfluidic channel ( \(\epsilon _{r}\) = 2.55, tan \(\delta\) = 0.002) over a metallic grounded LCP substrate ( \(\epsilon _{r}\) = 2.9, tan \(\delta\) = 0.0025) 10 , 32 . The soft lithographic processes can be used to fabricate microfluidic channel 5 , 10 , 33 . The microfluidic channel of PMMA elastomer can be sealed with a thin and flat sheet of LCP-based substrate layer 10 , 34 . The position of metallic liquid in the microfluidic channel can be chosen as per the need of the desired result. The position of metallic liquid in different locations leads to modification in antenna results in terms of frequency and/or radiation pattern. In the present work, the position of metallic liquid is created at the center of the microfluidic channel.
The injection of metallic liquid at a certain position in the microfluidic channel can be possible in the following way. Initially, a syringe can inject the metallic liquid into the PMMA channel to fill the microfluidic that defines the radiating element. A bidirectional micropump unit is needed to reconfigure the physical length of the antenna by retracting a portion of the metallic liquid volume. Physical displacement of metallic liquid can be achieved through microfluidic techniques like pumping or electrowetting 34 . Digital microfluidics is also a new consideration for the physical displacement of metallic liquid in microfluidic channel 35 . Another way to set the metallic liquid at a certain position in the microfluidic channel is by filling the empty sections with de-ionized water 34 . Since the total volume of water is very small, the frequency/radiation pattern modifications and efficiency reduction due to the water and losses are not significant 34 .
In addition to flexibility, another advantage of a metallic liquid antenna in a microfluidic channel is its reconfigurability. The properties of the metallic liquid, such as its volume and shape, can be adjusted to tune the antenna’s operating frequency. The movement of liquid inside the microfluidic channel in different locations can reconfigure the radiation pattern. The antenna can achieve reconfigurability by changing the metallic liquid configuration within the microfluidic channel.
Data availibility
All data generated or analyzed during this study are included in this manuscript.
Wang, C.-X. et al. On the road to 6g: Visions, requirements, key technologies, and testbeds. IEEE Commun. Surv. Tutor. 25 , 905–974 (2023).
Article Google Scholar
Bogale, T., Wang, X. & Le, L. Chapter 9 - mmwave communication enabling techniques for 5G wireless systems: A link level perspective. In mmWave Massive MIMO (eds Mumtaz, S. et al. ) (Academic Press, Cambridge, 2017).
Google Scholar
Shayea, I., Abd. Rahman, T., Hadri Azmi, M. & Islam, M. R. Real measurement study for rain rate and rain attenuation conducted over 26 GHz microwave 5G link system in Malaysia. IEEE Access 6 , 19044–19064 (2018).
Alzoubi, K. et al. Effect of lamination on the bending fatigue life of copper coated PET substrate. In Advances in Display Technologies; and E-papers and Flexible Displays , vol. 7956, 79560X. International Society for Optics and Photonics (SPIE, 2011).
So, J. et al. Reversibly deformable and mechanically tunable fluidic antennas. Adv. Funct. Mater. 19 , 3632–3637 (2009).
Article CAS Google Scholar
Materials for flexible and printed electronics. Materials Matter, Aldrich Materials Science Sigma-Aldrich Co. LLC .
Zou, M., Hu, Z., Hua, C. & Shen, Z. Design of plasmonic rectangular ribbon antenna based on graphene for terahertz band communication. Wiley Encycl. Electr. Electron. Eng. 15 , 1–23 (2016).
Kosta, Y. P., ChaIurvedi, B. K. & et al. A liquid patch microwave antenna. In Proceedings of NACONECS-1989, , Dept. of Electronics and Computer Engineering, University of Roorkee, India, Tata McGrawhill , 43–47 (1989).
Kosta, Y. P. Mercury patch micmwave antenna. In Space Applications Centre (ISRO), Proceedings of the International Conference on Smart Materials, Structures and Systems, Indian Institute of Science, Bangalore, India , 701–706 (1999).
Dey, A., Guldiken, R. & Mumcu, G. Microfluidically reconfigured wideband frequency-tunable liquid-metal monopole antenna. IEEE Trans. Antennas Propag. 64 , 2572–2576 (2016).
Article ADS MathSciNet MATH Google Scholar
Hayes, G. J., So, J.-H., Qusba, A., Dickey, M. D. & Lazzi, G. Flexible liquid metal alloy (egain) microstrip patch antenna. IEEE Trans. Antennas Propag. 60 , 2151–2156 (2012).
Article ADS Google Scholar
Morishita, A. M., Kitamura, C. K. Y., Ohta, A. T. & Shiroma, W. A. A liquid-metal monopole array with tunable frequency, gain, and beam steering. IEEE Antennas Wirel. Propag. Lett. 12 , 1388–1391 (2013).
Bo, G., Ren, L., Xu, X., Du, Y. & Dou, S. Recent progress on liquid metals and their applications. Adv. Phys. 3 , 412–442 (2018).
Dickey, M., Chiechi, R., Larsen, R., Weiss, E. & Whitesides, G. Eutectic gallium–indium (EGaIn): A liquid metal alloy for the formation of stable structures in microchannels at room temperature. Adv. Funct. Mater. 18 , 1097–1104 (2008).
Dash, S. & Patnaik, A. Material selection for THz antennas. Microw. Opt. Technol. Lett. 60 , 1183–1187 (2018).
Dash, S. & Patnaik, A. Advancements in terahertz antenna design and their performances. In Next Generation Terahertz Wireless Communication Networks (CRC Press, USA, Taylor and Francis Group, 2021).
Dash, S. & Patnaik, A. Performance of graphene plasmonic antenna in comparison with their counterparts for low-terahertz applications. Plasmonics 13 , 2353–2360 (2018).
Varnava, C. Liquid metals take stretchable circuits to new heights. Nat. Electron. 2 , 52 (2019).
Zou, M., Shen, Z. & Pan, J. Liquid antennas. Wiley Encycl. Electr. Electron. Eng. 1–23 (2016).
Sruti, A. N. & Jagannadham, K. Electrical conductivity of graphene composites with In and In-Ga alloy. J. Electron. Mater. 39 , 1268–1276 (2010).
Article ADS CAS Google Scholar
Novoselov, K. S. et al. Electric field effect in atomically thin carbon films. Science 306 , 666–669 (2004).
Article ADS CAS PubMed Google Scholar
Marlinda, A. R. et al. Graphene as a lubricant additive for reducing friction and wear in its liquid-based form. Lubricants 11 , 1–27 (2023).
Yang, W. & Wang, C. Graphene and the related conductive inks for flexible electronics. J. Mater. Chem. C 4 , 7193–7207 (2016).
Golovachev, Y., Etinger, A., Pinhasi, G. A. & Pinhasi, Y. Propagation properties of sub-millimeter waves in foggy conditions. J. Appl. Phys. 125 , 151612 (2019).
Kumar, S., Dixit, A. S., Malekar, R. R., Raut, H. D. & Shevada, L. K. Fifth generation antennas: A comprehensive review of design and performance enhancement techniques. IEEE Access 8 , 163568–163593 (2020).
Alibakhshikenari, M. et al. An innovative antenna array with high inter element isolation for sub-6 GHz 5G MIMO communication systems. Sci. Rep. 12 , 1–13 (2022).
Marasco, I. et al. A compact evolved antenna for 5G communications. Sci. Rep. 12 , 1–11 (2022).
Marasco Parchin, N. O. et al. An efficient antenna system with improved radiation for multi-standard/multi-mode 5G cellular communications. Sci. Rep. 13 , 1–15 (2023).
Hasan, M. M. et al. Gain and isolation enhancement of a wideband mimo antenna using metasurface for 5G sub-6 GHz communication systems. Sci. Rep. 12 , 1–17 (2022).
HFSS- High frequency structure simulator. ANSYS HFSS Version 21.
Gusynin, V., Sharapov, S. G. & Carbotte, J. P. Magneto-optical conductivity in graphene. J. Phys. Condens. Matter 19 , 026222 (2006).
Ling, K., Kim, H. K., Yoo, M. & Lim, S. Frequency-switchable metamaterial absorber injecting eutectic gallium-indium (EGain) liquid metal alloy. Sensors 15 , 28154–28165 (2015).
Article ADS CAS PubMed PubMed Central Google Scholar
Xia, Y. & Whitesides, G. M. Soft lithography. Angew. Chem. Int. Ed. 37 , 550–575 (1998).
Rodrigo, D., Jofre, L. & Cetiner, B. A. Circular beam-steering reconfigurable antenna with liquid metal parasitics. IEEE Trans. Antennas Propag. 60 , 1796–1802. https://doi.org/10.1109/TAP.2012.2186235 (2012).
Wan, Z., Zeng, H. & Feinerman, A. Reversible electrowetting of liquid-metal droplet. J. Fluids Eng. 129 , 388–394. https://doi.org/10.1109/TAP.2012.2186235 (2006).
Download references
Acknowledgements
This work has received funding from the European Research Council (ERC) under the European Union’s Horizon 2020 research and innovation programme (Grant Agreement No. 819819). It was also co-funded by the European Regional Development Fund and the Repulbic of Cyprus through the Research and Innovation Foundation, under the project CONCEPT/0722/0123.
Author information
Authors and affiliations.
Department of Electrical and Computer Engineering, University of Cyprus, Nicosia, Cyprus
Sasmita Dash, Constantinos Psomas & Ioannis Krikidis
You can also search for this author in PubMed Google Scholar
Contributions
All authors S.D., C.P., and I.K. contributed to the design and implementation of the research, analysis of the results, and the manuscript’s preparation.
Corresponding author
Correspondence to Sasmita Dash .
Additional information
Publisher's note.
Springer Nature remains neutral with regard to jurisdictional claims in published maps and institutional affiliations.
Rights and permissions
Open Access This article is licensed under a Creative Commons Attribution 4.0 International License, which permits use, sharing, adaptation, distribution and reproduction in any medium or format, as long as you give appropriate credit to the original author(s) and the source, provide a link to the Creative Commons licence, and indicate if changes were made. The images or other third party material in this article are included in the article’s Creative Commons licence, unless indicated otherwise in a credit line to the material. If material is not included in the article’s Creative Commons licence and your intended use is not permitted by statutory regulation or exceeds the permitted use, you will need to obtain permission directly from the copyright holder. To view a copy of this licence, visit http://creativecommons.org/licenses/by/4.0/ .
Reprints and permissions
About this article
Cite this article.
Dash, S., Psomas, C. & Krikidis, I. Selection of metallic liquid in sub-6 GHz antenna design for 6G networks. Sci Rep 13 , 20551 (2023). https://doi.org/10.1038/s41598-023-47870-7
Download citation
Received : 23 September 2023
Accepted : 19 November 2023
Published : 23 November 2023
DOI : https://doi.org/10.1038/s41598-023-47870-7
Share this article
Anyone you share the following link with will be able to read this content:
Sorry, a shareable link is not currently available for this article.
Provided by the Springer Nature SharedIt content-sharing initiative
By submitting a comment you agree to abide by our Terms and Community Guidelines . If you find something abusive or that does not comply with our terms or guidelines please flag it as inappropriate.
Quick links
- Explore articles by subject
- Guide to authors
- Editorial policies
Sign up for the Nature Briefing newsletter — what matters in science, free to your inbox daily.

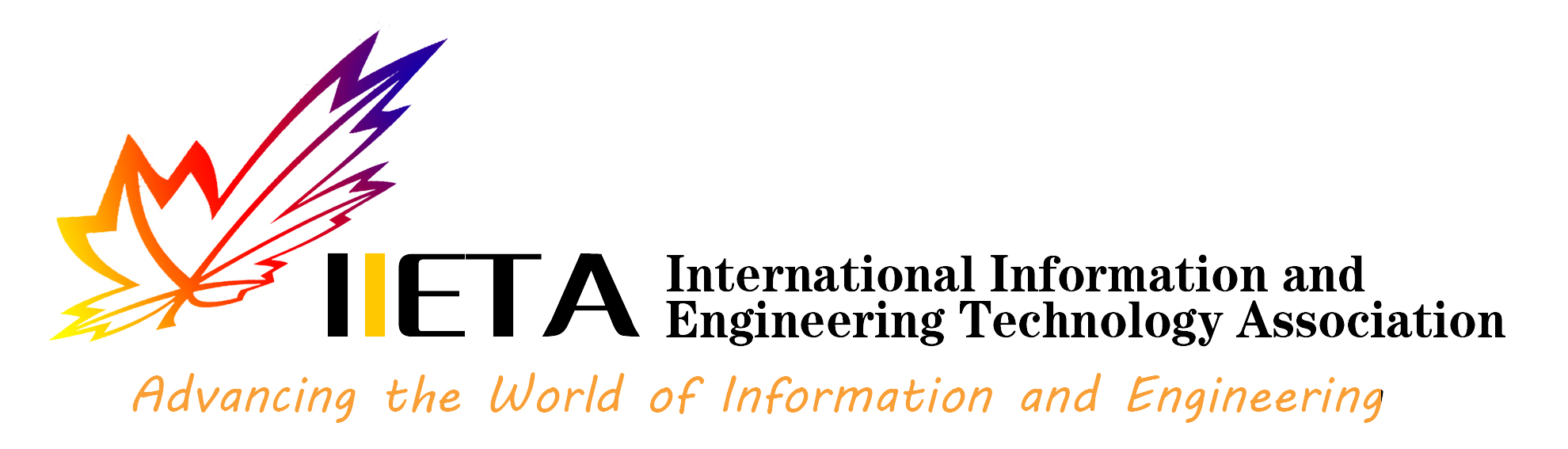
Search form
Design and study of a microstrip patch antenna for gps application.
Mouloud Ayad * | Kamel Saoudi | Turki E.A. Alharbi | Mohammed S. Alzaidi | Mourad Benziane | Souhil Mouassa | Sherif S.M. Ghoneim
© 2022 IIETA. This article is published by IIETA and is licensed under the CC BY 4.0 license ( http://creativecommons.org/licenses/by/4.0/ ).
OPEN ACCESS
A microstrip patch antenna for Global Positioning System (GPS) application is presented in this study. It has a rectangular shape with two notched slots. The notched slots are introduced to improve the adaptation between the microstrip line and the antenna. The antenna was designed and simulated by CST using FR-4 material as the substrate with relative permittivity of 4.3. The proposed microstrip antenna is designed to operate in the GPS band frequency from 1.555 GHz to 1.595 GHz. The performance analysis of the proposed antennas has been carried out in terms of return loss (dB), gain (dB), and VSWR.
microstrip patch antenna, GPS, return loss, VSWR
Telecommunications systems are very widely present in our current smart lifestyles. The Global Positioning System (GPS) is one of these systems. It is a satellite geographic positioning system used by various navigation systems. These localization systems have become essential to the tremendous demands for new services and applications in multiple civil and military domains. GPS is very widely used in several domains, such as in monitoring systems [1-6], intelligent systems [7-9], power management [10], and other applications. Moreover, GPS can provide real-time location information for an unlimited number of users. A satellite navigation system allows users equipped with a receiver to calculate and determine their position at any place on earth from the estimation of the distance which separates them from a minimum number of satellites. When we talk about GPS, we must systematically and specifically consider the equipment used to provide location. However, the GPS is not limited to this type of instrument, as it consists of three different elements called segments or sectors. The first segment of satellites is called the space segment. The second segment comprising the control station is called the control segment, and the last segment corresponding to the GPS receiver is called the user segment. In user equipment, we find the essential element of transmission. In the GPS systems, the antennas do the emission and reception of the data. These antennas are used at the reception level and are generally placed in land vehicles, aircraft, and ships to precisely locate their positions. It has advantageous characteristics (wide frequency band, security, and high speed). The antennas aim to perfect and improve several performances for all wireless communication systems [11-14]. Therefore, the antenna is a crucial element for coupling the device and the propagation medium. Hence, the design and proposition of a microstrip patch antenna for GPS application is a vital task.
Several microstrip patch antenna are presented in the literature for GPS applications [15-23].
Supriya and Rajendran [17] proposed an antenna for a GPS system based on an open-loop resonator, a split rectangular slot, and a stub at the non-radiating edge to generate three operating bands. Awais et al. [18] use four-element antenna array for the proposed antenna for a navigation satellite system. It is an assembled of 4 single patch antenna elements. Based on a circularly polarized dielectric resonator, Sharma et al. [19] present an antenna for GPS application that operates at 1.56 GHz. Lee et al. [20] proposed a patch antenna with a metallic reflector. This antenna is designed for GPS applications and operates at 1.575 GHz. The occupied volume by the antenna and the reflector is very extensive. With the addition of an artificial magnetic conductor plane, the proposed antenna [21] operates with a dual-band at 1.575 GHz for GPS and at 2.45 GHz for WLAN applications. Another antenna that operates in dual bands for GPS application is proposed by Zhong et al. [22]. The proposed antenna consists of two patches to generate two bands. Another antenna is proposed by Pourbagher et al. [23] with circular polarization. The structure of the antenna is composed of two orthogonally dipoles.
The main challenges in these proposed antennas are volume and occupied areas. Therefore, this paper aims to design, simulate, and analyze an antenna with a reduced volume destined for GPS application.
The rest of this paper is organized as follows: In section 2, the antenna design steps are presented. The results and discussion are given in Section 3. Finally, the last section provides the conclusion.
This part is devoted to studying the influence of the different parameters on the reflection coefficient S11 and the VSWR. A parametric study was conducted to obtain an effective and optimized final structure to understand the various parameters' influence. We use FR-4 material as the substrate, usually with relative permittivity equal to 4.3. The parameters to modify are the length (Lp) and the width (Wp) of the patch, the width of the microstrip line (Wf), the width (gpf), and the length (fi) of the notch, and the thickness of the substrate (hs). The proposed antenna structure and detailed configurations are illustrated in Figure 1.
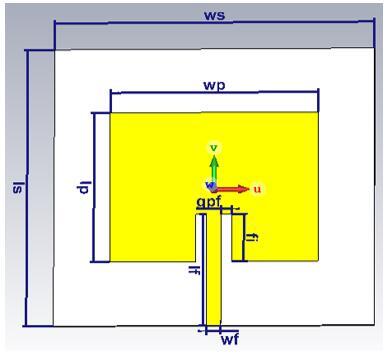
Figure 1. Antenna structure
This part will present the structure and simulation results of the patch antenna of rectangular shape fed by the microstrip line, and this is for a GPS application in a frequency band of value 1.575 GHz [20-23].
The sections below present the evolution steps of the design of the proposed antenna according to the following forms: the ground plane, the substrate, and the radiating patch. For this antenna, we have chosen a power supply by microstrip line. The parametric study justifies this choice to improve the GPS frequency band of [1.555-1.595] GHz.
2.1 Influence of the (Lp) patch length
The length of the patch has a vital role in determining the resonant frequency. This part will allow us to see the variation in the size of the patch (Lp). The results obtained are represented the Table 1.
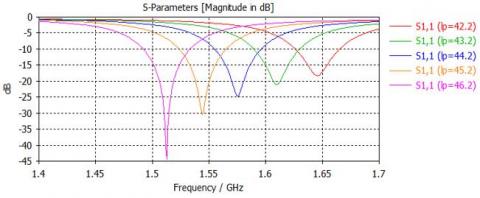
Figure 2. Influence of length (lp) by the contribution of reflection coefficient (S11)
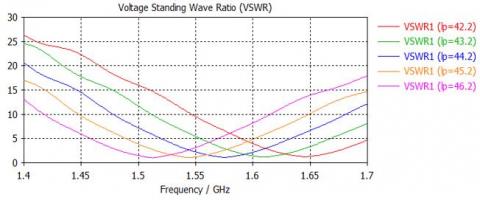
Figure 3. Influence of the length (lp) by the standing wave ratio VSWR contribution
Figure 2 and Figure 3 show the results of the influence of the length of the patch (Lp) by input reflection coefficient (S11) and the VSWR.
In Figure 3, we note that the length of the patch mainly affects the matching of the antenna. The patch size (lp) reduction leads to an increase in the resonant frequency and bandwidth. Also, from Figure 3 and Table 1, we observed that for all changes in length that we made, the VSWR remains less than 2 (VSWR ≤ 2), therefore, we can conclude that the length lp3= 44.2 mm is the best to improve the frequency band [1.555 to 1.595] GHz.
2.2 Influence of patch width Wp
The patch's parameters (the length (Lp), the permittivity (εr), and the height (hs) of the substrate) are fixed during the study. However, the parameter relating to the width of the patch varies continuously.
The results obtained are shown in Table 2.
The influence of length (Wp) by the contribution of reflection coefficient (S11) is given in Figure 4.
In the case of a variation in the width of the patch, presented in Table 2 and Figure 5, show us that the bandwidth and the resonant frequency register minor downward variations while the reflection coefficient varies.
According to Figure 5, we notice that the VSWR gives us values ≤ 2, so we conclude that the width wp3 = 58.5 mm is the best width to improve the frequency band [1.555-1.595] GHz.
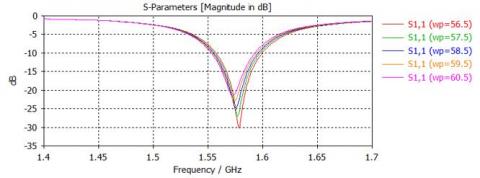
Figure 4. Influence of length (Wp) by the contribution of reflection coefficient (S11)
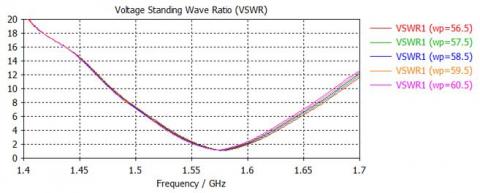
Figure 5. Influence of the width (Wp) by the standing wave ratio VSWR contribution
2.3 Influence of the thickness (hs) of the substrate
We will fix all the antenna parameters and change the value of the thickness (hs) of the substrate. The results obtained are identified in Table 3.
Figure 6 below clearly shows the variation of the thickness (hs) of the substrate on the parameter S11.
Table 1. Study the variation of the patch length (lp) on the antenna
Table 2. Study the variation of the patch width (wp) on the antenna
Table 3. Study the variation of the substrate thickness (hs) on the antenna
Table 4. Study the variation of the microstrip line width (wf) on the antenna
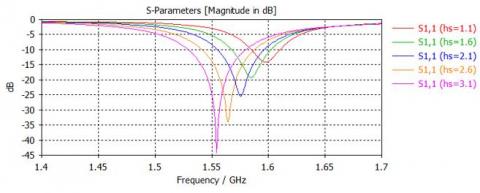
Figure 6. Influence of thickness (hs) by the contribution of reflection coefficient (S11)
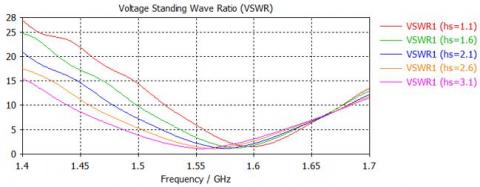
Figure 7. Influence of the thickness (hs) by the standing wave ratio VSWR contribution
Figure 6 shows that the bandwidth increases as the substrate thickness (hs) increase. Moreover, the resonant frequency decreases as the thickness (hs) grow. Therefore, the resonant frequency is inversely proportional to the substrate thickness (hs). On the other hand, the bandwidth is symmetrical to the thickness (hs) of the substrate.
The influence of the thickness (hs) of the substrate on VSWR is presented in Figure 7.
According to Figure 7 and the results of Table 3 obtained, the different thicknesses of the substrate (hs), the VSWR ≤ 2, so we conclude that the thickness hs3= 2.1 mm is the best to improve the frequency band [1.555-1.95] GHz.
2.4 Influence of the width of the micro ribbon line wf
By changing the width of the microstrip line (wf), we distinguish the different variables according to Table 4.
The influence of different widths line (wf) of the microstrip on the reflection coefficient (S11), and the VSWR are presented in Figures 8 and 9.
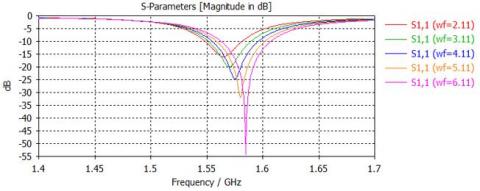
Figure 8. Influence of width (wf) by the contribution of reflection coefficient (S11)
According to the data in Table 4 and Figure 9, we note that with each increase in the microstrip line width (wf), the resonance frequency and bandwidth increase while the reflection coefficient changes.
According to Figure 9, we observe that for all the widths of the micro-strip line wf the VSWR ≤ 2, according to these results, we conclude that the width wf3= 4.11mm is the best to perfect the frequency band [1.555–1.595] GHz.
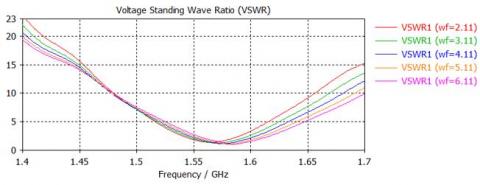
Figure 9. Influence of the width (wf) by the standing wave ratio VSWR contribution
2.5 Influence of the (gpf) width of the notch
We have found in the literature that adding a notch makes it better to fit the antenna fed by the microstrip line. The following Table 5 summarizes all of the notch width change results.
Table 5. Study the variation of the width of the notched (gpf) on the antenna
The notch width variation (gpf), in terms of the reflection coefficient S11 and VSWR, are shown in Figure 10 and Figure 11, respectively.
From the analysis of Figure 10, we find that the width (gpf) of the notch, for small values, the bandwidth decreases with the stability of the resonant frequency. On the other hand, when the value of (gpf) is zero, the adaptation between the microstrip line and the antenna is weak.
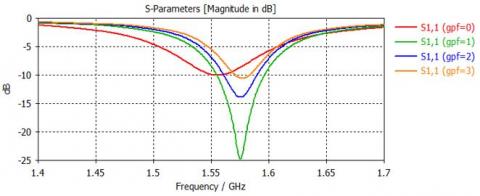
Figure 10. Influence of width (gpf) by input reflection coefficient (S11)
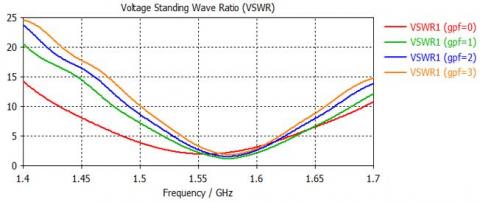
Figure 11. Influence of the (gpf) width by the standing wave ratio VSWR contribution
Figure 11 shows VSWR (dB) as frequency (GHz) function. Please note that our VSWR≤ 2 for all notch (gpf) widths used. Based on these results, we conclude that the width gpf2= 1 mm is better to improve the [1.555-1.595] GHz band.
2.6 Influence of the length (fi) of the notch
The notched band is recommended to improve the adaptation between the microstrip line and the antenna. The following table shows some results on the variation of the values of the notch length.
The next curve gives the results of the influence of the length (fi) of the notch concerning the reflection coefficient S11.
The curve above shows the results of the influence of the length (fi) of the notch concerning the VSWR.
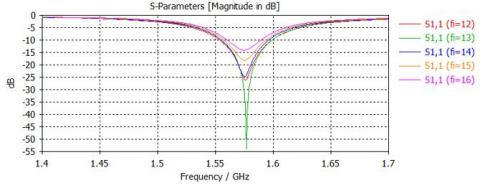
Figure 12. Influence of length (fi) by the contribution of reflection coefficient (S11)
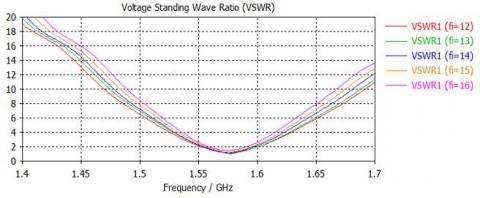
Figure 13. Influence of the length (fi) by the standing wave rate VSWR contribution
Table 6. Study the variation of the length of the notched (fi) on the antenna
Figure 12 indicates that the resonance frequency remains almost the same for different lengths with a very slight variation, while the level of S11 is varied.
On the other hand, through Figure 13 and Table 6, we noticed that by making all the changes related to the (gpf) length of the notch, the VSWR always remains less than or equal to 2 (VSWR≤2).
From the studies we have done, we note that for the resonant frequency equal to 1.575 GHz, we find that the impedance is Z = 50.7-0.31j, so we can say that when the fundamental part of impedance input reaches its maximum, the imaginary part vanishes.
We can deduce the final parameters to improve the frequency band; see Table 7 below.
After designing the antenna, we simulate the latter's operation, which was intended for the GPS application.
The results obtained by studying this antenna are represented in several parameters, the most important: the reflection coefficient (S11), VSWR, and the radiation pattern, which are shown in the figures below.
Figure 14 shows the result of the simulation of the reflection coefficient S11 (dB) as a function of frequency (GHz). We observed that the antenna showed a good adaptation in the band [1.555-1.595] GHz, so the reflection coefficient is less than -10dB, then the latter is the smallest at the resonance frequency of 1.575 GHz at - 24.804dB.
Figure 15 determines the result of the standing wave ratio VSWR as a function of frequency (GHz). The figure shows that VSWR ≤ 2 corresponds to S11 ≤ − 10 dB in the same frequency band (1.555-1.595) GHz.
CST software makes it possible to visualize the radiation diagram in gain and directivity after the simulation (Figures 16 (a) and (b)). We observe that the radiation diagram of this antenna is oriented in the Z direction. We note that the gain and directivity are positive when the antenna radiates on the 1.575 GHz frequency band.
Figure 17 shows the radiation patterns in two planes: E and H, for the antenna frequency, studied (1.575 GHz).
From Figure 17, the antenna radiates in the direction (θ= 0°). The angular widths are 96.9° and 71.9° in the E-plan and H-plane, respectively. The corresponding side lobe levels are -12.1 dB and -12.6 dB.
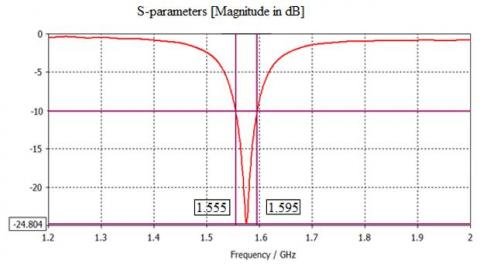
Figure 14. Reflection coefficient (S11) of the proposed antenna
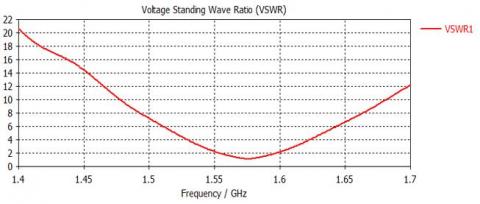
Figure 15. VSWR of the proposed antenna
Table 7. Size of the optimized antenna
Table 8. Comparison of the proposed antenna with related works
* The circular shape with D is the diameter (mm), and h is the thickness
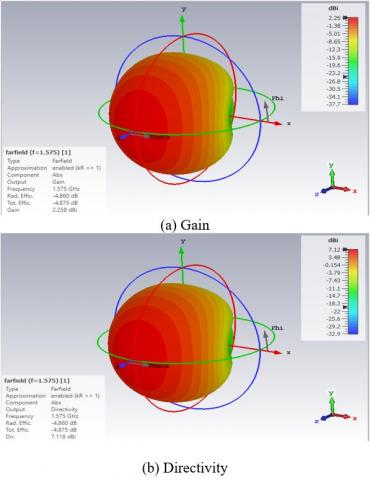
Figure 16. 3D radiation patterns of proposed antenna
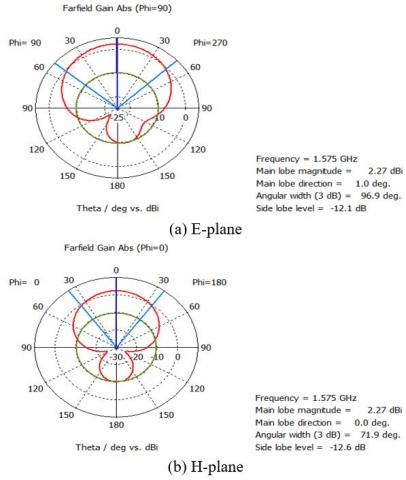
Figure 17. 2D gain radiation pattern
To give significance to the proposed antenna, a comparison with recently proposed antennas is necessary. The performance of the proposed antenna is compared with recently proposed antennas in the literature is given in Table 8.
The two sizes [19] are the antenna's size and the dielectric resonator. The two sizes are those of the antenna and the reflector [20].
According to Table 8, the proposed antenna has a good array area and good occupied volume.
A microstrip patch antenna has been proposed and investigated for GPS application. The proposed antenna has compact dimensions of 95×82×2.1 mm 3 . The antenna is designed to operate in the GPS band frequency from 1.555 GHz to 1.595 GHz and resonates at 1.575 GHz with a return loss of -24.804 dB. The material used for the substrate is FR-4, with relative permittivity of 4.3. The performance analysis of the proposed antennas has been tested by the obtained values of the return loss (dB), gain (dB), and VSWR. The obtained results verify the satisfactory performance of the proposed antenna for GPS application.
The following steps to deepen this study are the miniaturization of the antenna's dimensions in future work. In the second part, the antenna's operating frequency must be extended to multiband to support other ranges of applications.
This research was supported by the collaboration of University of Bouira, Algeria (PRFU N°: A10N01UN100120220001) and Taif University Researchers Supporting Project Number TURSP-2020/34, Taif University, Taif, Saudi Arabia.
[1] Zhang, C., Xue, W., Xin, Y. (2019). Design and application of an intelligent patrol algorithm for forest management and protection based on global positioning system. Ingénierie des Systèmes d'Information, 24(6): 597-602. https://doi.org/10.18280/isi.240606 [2] Bandyopadhyay, M., Mandal, N., Chattopadhyay, S., Roy, B. (2017). A novel GSM and GPS based vehicle security system. Advances in Modelling and Analysis D, 22(1): 62-76. https://doi.org/10.18280/ama_d.220105 [3] Plaza, J., Palacios, C., Abecia, J.A., Nieto, J., Sánchez-García, M., Sánchez, N. (2022). GPS monitoring reveals circadian rhythmicity in free-grazing sheep. Applied Animal Behaviour Science, 251: 105643. https://doi.org/10.1016/j.applanim.2022.105643 [4] Razin, M.R.G., Voosoghi, B. (2022). Modeling of precipitable water vapor from GPS observations using machine learning and tomography methods. Advances in Space Research, 69: 2671-2681. https://doi.org/10.1016/j.asr.2022.01.003 [5] Poliziani, C., Rupi, F., Schweizer, J. (2022). Traffic surveys and GPS traces to explore patterns in cyclist’s in-motion speeds. Transportation Research Procedia, 60: 410-417. https://doi.org/10.1016/j.trpro.2021.12.053 [6] Tran, Q.H., Fang, Y.M., Chou, T.Y., Hoang, T.V., Wang, C.T., Vu, V.T., Chen, M.H. (2022). Short-term traffic speed forecasting model for a parallel multi-lane arterial road using GPS-monitored data based on deep learning approach. Sustainability, 14(10): 6351. https://doi.org/10.3390/su14106351 [7] Hilal, H.A., Hilal, N.A., Hilal, T.A. (2022). Crowdsensing application on coalition game using GPS and IoT parking in smart cities. Procedia Computer Science, 201: 535-542. https://doi.org/10.1016/j.procs.2022.03.069 [8] Arshad, J., Rehman, A.U., Othman, M.T.B., Ahmad, M., Tariq, H.B., Khalid, M.A., Hamam, H. (2022). Deployment of wireless sensor network and IoT platform to implement an intelligent animal monitoring system. Sustainability, 14(10): 6249. https://doi.org/10.3390/su14106249 [9] Shi, Y., Yang, T., Zhang, S., Liu, L., Cui, Y. (2020). A Wi-Fi positioning system for material transport in greenhouses. Instrumentation Mesures Métrologies, 19(1): 65-72. https://doi.org/10.18280/i2m.190109 [10] Wei, X., Aman, M., Sikdar, B. (2021). Exploiting correlation among GPS signals to detect GPS spoofing in power grids. IEEE Transactions on Industry Applications, 58(1): 2022. https://doi.org/10.1109/TIA.2021.3131970 [11] El Yassini, A., Jallal, M.A, Ibnyaich, S., Zeroual, A., Chabaa, S. (2020). A miniaturized CPW-fed reconfigurable antenna with a single-dual band and an asymmetric ground plane for switchable band wireless applications. Traitement du Signal, 37(4): 633-638. https://doi.org/10.18280/ts.370412 [12] Gao, Y., Lu, H. (2019). A novel co-planar waveguide-fed direct current wide band printed dipole antenna. Traitement du Signal, 36(3): 253-257. https://doi.org/10.18280/ts.360308 [13] Farooq, U., Rather, G.M. (2019). Design and analysis of rectangular microstrip antenna (RMSA) for millimeter wave communication applications. Traitement du Signal, 36(5): 433-438. https://doi.org/10.18280/ts.360508 [14] Kumar, S., Dixit, A.S. (2021). A miniaturized CSRR loaded 2-element MIMO antenna for LTE band. Mathematical Modelling of Engineering Problems, 8(6): 984-988. https://doi.org/10.18280/mmep.080620 [15] Basit,A., Khattak, M.I., Sebak,R., Qazi, A., Telba,A. (2020). Design of a compact microstrip triple independently controlled pass bands filter for GSM, GPS and WiFi applications. IEEE Access, 8: 77156-77163. https://doi.org/10.1109/ACCESS.2020.2989377 [16] Liu, H., Shi, M., Fang, S., Wang, Z. (2020). Design of low-profile dual-band printed quadrifilar helix antenna with wide beamwidth for UAV GPS applications. IEEE Access, 8: 157541-145548. https://doi.org/10.1109/ACCESS.2020.3018906 [17] Supriya, A.S., Rajendran, J. (2017). A low cost tri-band microstrip patch antenna for GPS application. IEEE in 2017 Progress in Electromagnetics Research Symposium-Fall (PIERS-FALL), pp. 60-65. https://doi.org/10.1109/PIERS-FALL.2017.829311 [18] Awais, M., Madni, A., Khan, W.T. (2022). Design of a compact high isolation 4-element wideband patch antenna array for GNSS applications. IEEE Access, 10: 13780-13786. https://doi.org/10.1109/ACCESS.2022.3147600 [19] Sharma, A., Das, G., Gupta, S., Gangwar, R.K. (2020). Quad-band quad-sense circularly polarized dielectric resonator antenna for GPS/CNSS/WLAN/WiMAX applications. IEEE Antenna and Wireless Propagation Letter, 19(3): 403-407. https://doi.org/10.1109/LAWP.2020.2969743 [20] Lee, S., Yang, Y., Lee, K.Y., Hwang, K.C. (2020). Dual-band circularly polarized annular slot antenna with a lumped inductor for GPS application. IEEE Transaction on Antennna and Propagation, 68(12): 8197-8202. https://doi.org/10.1109/TAP.2020.2997990 [21] Joshi, R., Hussin, E.F.N.M., Soh, P.J., Jamlos, M.F., Lago, H., Al-Hadi, A.A., Podilchak, S.K. (2020). Dual-band, dual-sense textile antenna with AMC backing for localization using GPS and WBAN/WLAN. IEEE Access, 8: 89468-89478. https://doi.org/10.1109/ACCESS.2020.2993371 [22] Zhong, Z.P., Zhang, X., Liang, J.J., Han, C.Z., Fan, M.L., Huang, G.L., Yuan, T. (2019). A compact dual-band circularly polarized antenna with wide axial-ratio beamwidth for vehicle GPS satellite navigation application. IEEE Transactions on Vehicular Technology, 68(9): 8683-8692. https://doi.org/10.1109/TVT.2019.2920520 [23] Pourbagher, M., Nourinia, J., Ghobadi, C. (2020). Circularly polarized printed crossed-dipole antenna using branch-line feed network for GPS applications. AEU-International Journal of Electronics and Communications, 120: 153226. https://doi.org/10.1016/j.aeue.2020.153226
Phone: + 1 825 436 9306
Email: [email protected]
Subscription
Language support
Please sign up to receive notifications on new issues and newsletters from IIETA
Select Journal/Journals:
Copyright © 2024 IIETA. All Rights Reserved.

An official website of the United States government
The .gov means it’s official. Federal government websites often end in .gov or .mil. Before sharing sensitive information, make sure you’re on a federal government site.
The site is secure. The https:// ensures that you are connecting to the official website and that any information you provide is encrypted and transmitted securely.
- Publications
- Account settings
Preview improvements coming to the PMC website in October 2024. Learn More or Try it out now .
- Advanced Search
- Journal List
- Micromachines (Basel)
- PMC10385670

A Comprehensive Review of In-Body Biomedical Antennas: Design, Challenges and Applications
Khaled aliqab.
1 Department of Electrical Engineering, College of Engineering, Jouf University, Sakaka 72388, Saudi Arabia
Iram Nadeem
2 Department of Information Engineering and Mathematics Science, University of Siena, 53100 Siena, Italy; [email protected]
Sadeque Reza Khan
3 Institute of Sensors, Signals and Systems, School of Engineering and Physical Sciences, Heriot-Watt University, Edinburgh EH14 4AS, UK

Associated Data
No new data were created in this research.
In-body biomedical devices (IBBDs) are receiving significant attention in the discovery of solutions to complex medical conditions. Biomedical devices, which can be ingested, injected or implanted in the human body, have made it viable to screen the physiological signs of a patient wirelessly, without regular hospital appointments and routine check-ups, where the antenna is a mandatory element for transferring bio-data from the IBBDs to the external world. However, the design of an in-body antenna is challenging due to the dispersion of the dielectric constant of the tissues and unpredictability of the organ structures of the human body, which can absorb most of the antenna radiation. Therefore, various factors must be considered for an in-body antenna, such as miniaturization, link budget, patient safety, biocompatibility, low power consumption and the ability to work effectively within acceptable medical frequency bands. This paper presents a comprehensive overview of the major facets associated with the design and challenges of in-body antennas. The review comprises surveying the design specifications and implementation methodology, simulation software and testing of in-body biomedical antennas. This work aims to summarize the recent in-body antenna innovations for biomedical applications and indicates the key research challenges.
1. Introduction
Body-centric communication system (BWCS) is an emerging technology referring to human self and human-to-human networking, which uses implantable and wearable sensors [ 1 ]. BWCS is a combined field of wireless body area networks (WBANs), wireless personal area networks (WPANs) and wireless sensor networks (WSNs). It is also classified as off-body, on-body and in-body communication, as shown in Figure 1 . On-body communication is the communication between different wearable devices. The communication between an outside and an on-body device is designated as off-body communication [ 2 ]. In-body communication system means the communication of implantable devices and sensors inside the body with an external device or communication with another implant. In-body biomedical devices (IBBDs) are designed to monitor physiological data inside the human body and provide key support to improve the quality of life through disease prevention, therapy and diagnosis, such as drug delivery system, neurostimulators, bone growth stimulators, and treatment of numerous severe conditions in the medical profession [ 3 ]. Wireless IBBDs are divided into implantables, ingestibles and injectables based on the way they are inserted into the human body [ 4 ]. Specifically, implantable devices are the most common type of IBBDs sited inside the human body through a surgical operation [ 5 ]. In the last decade, implants have advanced from bulky pacemakers to micro-sized deep brain implants [ 6 ]. Ingestible devices are generally capsule-shaped devices, which are ingested and swallowed, similar to regular pills [ 7 ]. The most conventional ingestible device is the wireless endoscopic capsule, which was originally discovered in the year 2000 [ 8 ]. Currently, wireless ingestible capsules are equipped with cutting-edge abilities, which can also monitor the side effects of pharmaceuticals [ 9 ].

Types of BWCS.
Lastly, injectable devices [ 10 ] are micro-sized devices, which can be injected into the human body using needles. Recently, these devices have been commonly used for important sensing and neurostimulation applications [ 10 ]. The primary components of an IBBD are the antenna, battery, the processing system and sensors [ 11 ]. The reliability and strength of the wireless link between the external and internal device largely depend on the antenna mounted on an IBBD. Therefore, the antenna is a key construction block of an IBBD, as the primary working requirement of signal reception and transmission depends mainly on the performance of the antenna. Furthermore, the overall size and weight of the IBBD can also be affected by it.
In IBBDs, the antenna is primarily used for wireless communication and data transfer [ 12 , 13 ], wireless power transfer (WPT) [ 14 ] and sensing [ 15 , 16 ], which lead to a wide range of medical applications, including continuous pressure measurements, dental antenna for remote healthcare, intracranial pressure monitoring, glucose level check, insulin pump, radiometer/heating therapy, pacemaker connection, endoscopic capsule and blood pressure measurements [ 17 ].
The design of an in-body antenna is challenging, as it is mostly situated in electromagnetically harsh and lossy environments inside the human body. The electromagnetic (EM) wave passing through the lossy heterogeneous tissue inside the human body can cause significant absorption of most of the antenna radiations [ 18 ]. Such inhomogeneous human organ structure is the primary reason for the impedance mismatch, which makes the radiation performance inefficient. This also affects the antenna efficiency significantly [ 19 ]. The powering of an in-body antenna attached to an IBBD inside the human body is another key research challenge. The commonly used batteries in IBBDs are bulky in size with limited capacity and can complicate the system design process [ 20 ]. Recently, in-body antennas for WPT have become a great research interest [ 14 ]. WPT necessitates an appropriate selection of the frequency band, which is a vital component of the in-body antenna system for biomedical applications. The operation frequency band of an in-body antenna must avoid EM interference with the current terrestrial frequency bands [ 21 ]. Furthermore, the fabrication and testing of such miniaturized in-body antennas inside the human body are extremely challenging due to the inadequately accredited animal laboratories, along with major health and safety related issues [ 22 , 23 ].
The growing research in this area demands a comprehensive overview in order to acquaint the new researchers and antenna designers with the state of the art and current developments. This review work aims to describe the design specifications, implementation and testing techniques, challenges and different applications of in-body antennas. Following the Introduction, the paper is divided into six sections. Section 2 briefly highlights the design specifications of in-body antennas. Section 3 explains the in-body antenna design, manufacturing and testing process. Section 4 outlines the different challenges in the development of in-body antennas. Section 5 details the different antenna types being used in different IBBD applications and compares their performances critically. Section 6 briefly discusses the limitations of the current in-body antenna designs and indicates future research scopes. Finally, the conclusions are drawn in Section 7 .
2. Design Specifications
2.1. operation frequency bands.
Figure 2 shows different frequency bands allocated for in-body antennas. The choice of an operation frequency for IBBDs involves several trade-offs. Generally, lower frequencies are attractive and commonly used, as they facilitate lower loss of the biological tissue medium, which can lead to higher efficiency and better tissue safety. However, lower frequencies have the drawback of limiting the communication speed and requiring larger antenna size. In contrast, higher frequency bands provide high data rates and miniaturization at the cost of lower tissue safety. In Figure 2 , frequencies below 100 kHz are allocated for short-range inductive IBBDs by the Federal Communication Commission (FCC) in the United States (US) for lower power and data transmission [ 21 ]. Medical micropower networks (MMNs) are another FCC-approved short-range frequency band with a 24 MHz spectrum from 413 to 457 MHz. Furthermore, Wi-Fi (902–928 MHz), Bluetooth (2.40–2.483 GHz) and Zigbee (5.725–5.850 GHz) are designated for short-range digital modulation communication for IBBDs by the FCC in the US.

Frequency bands for in-body antennas.
In Europe, the Electronic Communication Committee (ECC) allocated the 430 to 440 MHz band for ultra-low-power wireless medical capsule endoscopy (ULP-WMCE) and the 2.483 to 2.50 GHz band for active medical implants (AMI). The Medical Device Radio Communication Service (MedRadio) band of 401–406 MHz [ 4 ], Medical Implant Communication System (MICS) band of 402–405 MHz [ 24 ] and Industrial, Scientific and Medical (ISM) band of 13.56 MHz, 433–434 MHz, 902–908 MHz, 2.40–2.48 GHz and 5.717–5.875 GHz [ 25 ] are accepted worldwide for IBBD application.
2.2. Miniaturization
Antenna miniaturization is a key specification for IBBDs. In MedRadio, MICS and common ISM bands, the effective size of the in-body antenna at the desired resonance frequency becomes significantly larger. This can create difficulties during the implantation process of IBBDs in human tissue [ 2 ]. Therefore, the size of in-body antennas is considered very crucial, and miniaturization techniques, such as changing the physical properties of the structure, varying the material characteristics or introducing supplementary elements, are used to solve these kinds of challenges.
2.2.1. High Permittivity Dielectric Substrate or Superstrate
High permittivity substrate/superstrate material can shift the antenna resonant frequency near to the lower frequency band, which shortens the operation wavelength [ 2 ]. This simple technique can provide a higher degree of miniaturization. Some of the materials, which are generally used as a dielectric substrate for in-body antennas, include alumina ceramic (relative permittivity, ε r = 9.4) [ 26 , 27 ] and Rogers 3210 [ 28 ], 3010 [ 29 ], 6002 [ 30 ] with ε r = 10.2. A substantial reduction in effective antenna length is realized by using MgTa 1.5 Nb 0.5 O 6 as a dielectric substrate, with the dielectric constant ε r = 28 [ 31 ]. However, this method results in a significant level of surface wave excitation within the substrate. This results in lower bandwidth and a decrease in overall radiation efficiency [ 32 ]. The higher cost of these materials is another issue.
2.2.2. Path Lengthening of Current Flow
By modifying the physical properties of an in-body antenna, it is possible to attain a prolonged path of effective current flow. This shifts the resonant frequency to a lower band, resulting in significant size reduction of the antenna [ 2 , 17 ]. Numerous design techniques are considered for this purpose, such as meandered [ 33 ], spiral [ 33 ], hook slotted [ 3 ], waffle type [ 30 ] and radiator staking methods [ 27 ]. This technique can suffer from higher ohmic loss, resulting in lower radiation efficiency [ 32 ].
2.2.3. Impedance Matching with Loading
In-body antennas commonly require the matching of impedance at the anticipated frequency of operation using loading techniques. The loading can possibly be inductive or capacitive, which can effectively minimize the imaginary part of the impedance by nullifying the effect of reactance, helping in size reduction. In Ref. [ 29 ], a circularly polarized implantable patch antenna was presented, where the size was reduced due to the use of capacitive loading compared to traditional square patch antenna. However, the impedance matching of a high-quality-factor ( Q -factor) in-body antenna can lead to performance issues and may require a separate compensation network. This can also reduce the bandwidth of the antenna.
2.2.4. Pin Shorting
Introducing a shorting pin between the patch planes and the ground increases the effective size of the antenna, resulting in a reduction of the essential physical dimensions of it for an explicit frequency of operation [ 2 , 3 ]. This is a similar technique, where the ground plane doubles the height of a monopole antenna. Therefore, it generally produces a planar inverted-F antenna (PIFA) with identical resonance performance, similar to a double-sized antenna deprived of the shorting pin [ 34 ]. However, it can cause a reduction in antenna aperture, resulting in a significant decrease in antenna directivity, which can affect the effective gain of the antenna directly [ 32 ].
2.2.5. High Frequency Band
The use of a higher frequency band can reduce the size of the in-body antenna significantly. Higher frequencies of operation have shorter wavelengths, which leads to a decrease in antenna size [ 2 , 17 ]. Alternatively, if the antenna size is reduced, the resonant frequency of the antenna will move to a higher band and vice versa. Higher frequencies with a wide bandwidth are also desirable for better data communication [ 35 ]. However, they suffer from higher tissue attenuation and loss compared to lower frequencies, which affect the overall in-body antenna performance by inducing more losses [ 36 ]. It is also necessary to maintain the operation frequency band specification, as described in Section 2.1 .
2.2.6. Modification of Ground Plane
In-body antennas can also be miniaturized by modifying their ground plane. Generally, the model of an in-body antenna considers infinite ground plane. In practice, the ground plane is designed as finite. For large-scale miniaturization, the size of the ground plane is further reduced in a way where, at times, it is slightly larger than the dimensions of the patch [ 32 ]. Refs. [ 37 , 38 , 39 ] present an analysis of truncated ground planes. Such miniaturization can be achieved by introducing a slot in the ground plane, which can alter the return path of the current to slow down the current flow. This causes a phase shift of the displacement of the current from one edge of the slot to the other, resulting in a smaller antenna size [ 40 ]. However, such in-body antenna has a reduced polarization concentration, and reducing the size of the ground plane can also affect input impedance. Furthermore, the edge diffraction due to ground plane modification can generate significant back lobe radiation, resulting in a reduction in front-to-back ratio [ 32 ].
2.2.7. Use of Metamaterial
Metamaterials are defined as artificially engineered materials, which are designed to provide material properties that are not commercially available to satisfy any extraordinary conditions [ 41 ]. They can also be engineered to achieve materials with close-to-zero values of permittivity, negative permittivity or permeability, or simultaneous negative permittivity and permeability. Therefore, they can dramatically reduce the in-body antenna size and can also improve its bandwidth and gain [ 42 ]. In Ref. [ 43 ], a circularly polarized in-body antenna could achieve 84% size reduction by using a metamaterial design. Although the use of metamaterials has been effective in reducing antenna size, there is a substantial cost in terms of using a complex material, significantly narrow operating bandwidths and lower radiation efficiencies [ 32 ]. Additionally, in metamaterial-based miniaturization methods, substantial care must be taken with the analytical models, which are used for the analysis. These analytical models typically ignore the polarization of the field, which might cause different behaviors as compared with the regular incidence or non-polarized models commonly used for analysis to calculate the effective medium properties.
2.3. Wireless Link Consideration
Figure 3 shows a generalized wireless communication link between an external device and an IBBD with a transceiver system and an antenna on each side.

Wireless communication link between external device and IBBD.
The wireless communication link can be classified as near field and far field. The near field technique includes inductive coupling [ 14 ]. It was the technology integrated into the first wireless implants at an operation frequency of 20 MHz or lower [ 4 ]. This technology established the use of inductors within IBBDs and external devices, which were located in close proximity to initiate wireless communication through coupling. The link design strategies for inductively coupled IBBDs are explained broadly in Refs. [ 14 , 44 ]. In far field, the link power budget can be written as [ 29 ]
where C / N 0 is the ratio of the carrier power and noise density; P t , G t , L f , G r , B r , G c , G d and E b / N 0 are the transmit power, transmit antenna gain, path loss (free space), receiver antenna gain, receiver bit rate, receiver coding gain, receiver deterioration and the ratio of energy per bit and noise density, respectively. The path loss can be calculated according to the free-space reduction in signal strength with the distance d between the transmitter and receiver as [ 29 ]
Furthermore, the impedance mismatch loss can be calculated as
where Γ is the appropriate reflection coefficient. The loss in human body is not considered in Equation (1), and this will be explained further in Section 4 , where different challenges for in-body antenna design are described.
2.4. Powering
IBBDs have been conventionally powered via batteries [ 11 , 45 ]. However, integration of the batteries can upsurge the size of the IBBDs, raising biocompatibility and patient safety related concerns. This also necessitates the requirement for frequent battery replacement and/or recharging due to short lifetime. Therefore, the research on battery-less techniques, such as energy harvesting and WPT, for IBBDs is becoming necessary. Energy harvesting technologies involve harvesting the power from environmental or human bodily sources. The motion of the tissue and heartbeat [ 46 ], body thermal gradients [ 47 ], human movement and motion [ 48 ], and glucose oxidization [ 49 ] are some of the mechanisms used in the past to harvest the energy for IBBDs. Different WPT techniques and their design methodologies for IBBDs are explained in Ref. [ 14 ]. Widespread research has been carried out in the last decade to improve the efficiencies of the aforementioned approaches and develop them to be used for powering IBBDs.
2.5. Biocompatibility
In-body antennas are installed in human bodies, so they must have biocompatible properties in order to satisfy patient safety. If in-body antennas are directly embedded into the human body, the body is short circuited due to the fact that human tissues are conductors. Therefore, as a measure to prevent such undesirable short circuit cases, biocompatibility becomes necessary for extended-term implantation of in-body antennas. Two types of methods are mostly proposed for biocompatibility issues of implantable antennas [ 3 ]. The first approach is to use a biocompatible substrate for antenna manufacturing, and the second approach is to cover the implantable antenna with a thin coating layer of biocompatible low-loss material. The biocompatible superstrate materials proposed for in-body antennas are Teflon, MACOR and Ceramic Alumina [ 34 ]. However, it is problematic to drill and assemble round cuts in ceramic substrates [ 50 , 51 ]. The materials proposed for antenna coating are PEEK [ 52 ], Zirconia [ 53 ], biomedical-grade-based Silastic MDX-4210 elastomer [ 51 ]. A significantly slim layer of low-loss biocompatible material coating increases the properties of biocompatibility in in-body antennas. However, a cautious design of the in-body antenna is required to avoid any performance dependencies related to the thickness of the biocompatible layer [ 54 ]. An improvement in the biocompatibility of in-body antenna coating Perylene C material on both sides of the antenna is also proposed in Ref. [ 55 ]. The electromagnetic properties of Zirconia make it a better contender for bio-encapsulation [ 3 ]. Its significantly lower loss tangent and higher permittivity value help decrease the power loss by accumulating the near field of the antenna inside the capsulation. Additionally, the benefit of PEEK and Silastic MDX-4210 is that they offer simple fabrication processes and are easy to handle.
2.6. Safety Consideration
The maximum allowable power incident in the in-body antenna is limited by issues related to patient safety. The specific absorption rate (SAR), which represents the amount of energy deposited per unit mass of tissue, is usually accepted as the most suitable scientific measure in compliance with international guidelines. The IEEE C95.1-1999 standard confines the average SAR over any 1 g of tissue in the shape of a cube to less than 1.6 W/kg (SAR 1g, max ≤ 1.6 W/kg) [ 56 ], which is followed by the FCC in the US. The international commission on non-ionizing radiation protection (ICNIRP) standardizes the limit of SAR averaged over 10 g of contiguous tissue to be less than 2 W/kg [ 57 ]. To comply with ICNIRP guidelines, the IEEE C95.1-2005 standard limits the average SAR over any 10 g of tissue in the shape of a cube to less than 2 W/kg (SAR 10g, max ≤ 2 W/kg), which is followed by the European Union [ 58 ].
3. Antenna Design, Manufacture and Testing
Figure 4 shows the generalized steps for designing, manufacturing and testing in-body antennas. In the first step, the researchers benchmark the antenna parameters based on the specifications provided in Section 2 . These parameters are then used to generate an analytical model, which leads to analysis and simulation in programming and numeric computing platforms, such as MATLAB [ 44 , 59 , 60 , 61 , 62 , 63 , 64 ]. This is an important step of optimizing the antenna to accomplish the best performance [ 65 ].

Generalized steps for designing, manufacturing and testing in-body antennas.
In the next step, the data generated from the analytical model are used to build a 3D antenna simulation environment using electromagnetic (EM) software, such as Ansys high frequency structure simulator (HFSS) [ 66 ] https://www.ansys.com/en-gb/products/electronics/ansys-hfss (accessed 15 May 2023), Dassault Systems CST microwave suite [ 67 ] https://www.3ds.com/products-services/simulia/products/cst-studio-suite/ (accessed 15 May 2023), finite difference time domain (FDTD) [ 68 ] https://optics.ansys.com/hc/en-us/articles/360034914633-Finite-Difference-Time-Domain-FDTD-solver-introduction (accessed 15 May 2023), Altair FEKO [ 69 ] https://altair.com/feko (accessed 16 May 2023) and COMSOL [ 64 ] https://www.comsol.com/rf-module (accessed 16 May 2023). Furthermore, Ansys Maxwell 3D [ 70 ] and Dassault Systems Simulia are low frequency solvers used to design in-body antennas with lower MHz to kHz frequency ranges. The analytical model and EM software simulation results are compared to verify and confirm the antenna parameters before manufacturing in-body antennas.
Detailed methodology of the manufacturing process of an in-body patch-type planar antenna has been outlined in Ref. [ 65 ]. In antenna manufacturing, a photolithography mask is first produced to confirm the antenna geometry, including antenna layers, which are going to be stacked on the plane. In the next step, the antenna layers are etched according to the antenna geometry using the photolithography mask. Furthermore, the lower substrate comprises the ground and lower patch; the upper substrate comprises the upper patch; and the superstrate is positioned on the top. Additionally, a circular-shaped hole is etched as per the patch geometry, where four pins are located at the base of the mask. Afterward, all the layers are machined to the circular format, and the layers of the antenna are positioned in a straight line.
This process must be conducted without putting much more mechanical stress on the antenna. Finally, the layers of the antenna are organized in a mountain format and glued to attach all the layers in case of a multi-layered structure. This step is not required for single-layer microstrip patch planar antenna. In the next step, the shorting pin is attached to the ground plane and lower patch. Therefore, the outer conductor of the co-axial feeding point is connected to the ground. Furthermore, the inner conductor is soldered to the lower and upper patch. This methodology is commonly used in research laboratories to validate the antenna parameters with respect to simulation. However, low-temperature ceramic Co-fire (LTCC) is a popular method used in industrial manufacturing. Helical antennas built with conductive wires are generally wound by hand and coil-winder-machined with a counter for laboratory testing and industrial manufacturing, respectively. The manufactured antenna is first characterized in the air, and the measured results are compared with the EM software simulation outputs. In case of a major discrepancy, the researchers go back to the previous step and reiterate it, as shown in Figure 4 . Otherwise, the in-body antenna is tested inside phantom (in vitro) and animal tissue (in vivo) successively.
3.1. Testing of In Vitro Antenna
The manufactured in-body antenna is verified in the in vitro antenna testing procedure using an artificially built biological environment or phantom [ 65 ]. The biological tissue properties (relative permittivity and electrical conductance) of different parts of the body at different operating frequencies are provided in the Foundation for Research on Information Technologies in Society (IT’IS) website, which was established through the resourcefulness and support of the Swiss Federal Institute of Technology (ETH) in Zurich [ 71 ]. Before preparing the phantom of a particular body part, it is necessary to know its permittivity and electrical conductance at the in-body antenna operation frequency. Low-frequency band liquid phantom, as shown in Figure 5 , is investigated in Refs. [ 70 , 72 ], where purified water, polyethylene powder and NaCl are used as the main material, relative permittivity and conductivity generation material, respectively. Several works presented the investigation of the phantom in the ISM and MICS bands [ 13 , 28 , 73 , 74 ]. In Ref. [ 13 ], a gel type phantom imitating the properties of muscle tissue is built with hydrophilic organic powder and degassed water, as shown in Figure 6 .

Liquid phantom for capsule localization investigation [ 70 ]. Reproduced with permission from an open-access article from IEEE Access (CC-BY).

( a ) Phantom setup. ( b ) Indentation of capsule in phantom [ 13 ]. Reproduced with permission from an open-access article from IEEE (CC-BY).
A traditional method of measuring the tissue properties of a phantom is the co-axial probe, where a dielectric probe kit, such as SPEAG DAK 3.5, along with a vector network analyzer, such as Agilent 8753ES, are used [ 75 ]. An alternative method utilized in the literature involves a dielectric resonator in close contact with the tissue [ 76 ]. These measurement techniques utilize the input reflection coefficient to calculate the material dielectric properties. Measurement uncertainty is significant for higher permittivity values, as there is less change in the measured reflection coefficient for discrepancies in material permittivity [ 75 ].
3.2. Testing of In Vivo Antenna
In vitro study is commonly carried out in an artificial biological environment, which cannot confirm the stability of the implanted antenna system because of the lack of dynamic illustration of a real biological environment in the in vitro study [ 51 ]. Therefore, the testing of in-body antenna in a real biological environment (in vivo) is commonly suggested after in vitro testing. Before implantation of the in-body antenna prototype inside the biological body, it is necessary to ensure that the temperature of the testing environment is below 100 °C. Generally, the in-body antenna itself generates heat up to 60 °C because of the battery and other internal system devices. Furthermore, the in-body antenna must be insulated by using biocompatible material to protect the antenna system from coupling loss, as described in Section 2.5 . Figure 7 and Figure 8 [ 23 , 77 ] illustrate the in vivo testing of the glucose monitoring implantable antenna in a rat and monitoring of blood pressure of the left ventricle, respectively.

Multi-layer implantable antenna measurement for continuous glucose monitoring. ( a ) A sensor implanted in a rat, ( b ) Experimental setup [ 77 ]. Reproduced by courtesy of the Electromagnetics Academy.

Anesthetized mixed landrace pig with ( a ) exposed left ventricular (LV) apex, ( b ) implanted wireless pressure sensor, ( c ) catheter-tip transducer and ( d ) chest spreader [ 23 ]. Reproduced with permission from an open-access article from Biomedical Microdevices (CC-BY).
4. Challenges That Influence the Design of In-Body Antennas
The development of in-body antennas faces numerous design challenges. Miniaturizing the effective electrical size of an antenna leads to a reduction in its electromagnetic performance [ 78 ]. Furthermore, it is necessary to consider some important factors to ensure patient safety during the design phase of in-body antennas. First, the in-body antenna is required to be biocompatible, and the SAR must be controlled within the standard limit. This section describes the factors that influence the design specifications of in-body antennas.
4.1. Effect of Tissue Diversification
The propagation of a radio wave through the biological tissue is more complex compared to wave propagation in a free space due to the lossy property of the biological tissue causing absorption. Absorption in a radio wave is primarily characterized by considering the permeability, permittivity and conductivity parameters of the medium. An EM wave propagating in the positive Z -direction is defined as [ 79 ]
where E and γ are the complex amplitude of the wave in the z -direction and the complex propagation constant, respectively. γ is defined as [ 79 , 80 ]
The constant increase in γ leads to attenuation of the electromagnetic wave inside the inhomogeneous region, where µ = µ r µ 0 = µ 0 defines the permeability of the medium, as for biological materials µ r = 1. However, the relative permittivity ε r (where ε = ε 0 ε r ) of human body tissue is a complex frequency-dependent parameter, as the conductivity σ is not zero. The dielectric properties of the human tissue can be obtained from its relative complex permittivity as [ 81 ]
where ε r ′ and ε r ″ are the real and imaginary parts of relative complex permittivity. The imaginary part of relative complex permittivity can be determined from the angular frequency ω and conductivity σ by
Furthermore, the loss tangent, tan δ , which is a measure of how lossy the human body can be, is calculated as follows:
Therefore, as per Equation (7), greater conductivity demonstrates a higher relative complex permittivity value. Furthermore, the increase in the frequency leads to a lower value of imaginary relative complex permittivity in a lossy medium. As a result, the increase in the loss tangent makes the medium significantly lossy. In brief, the complex propagation constant depends on three parameters: permittivity, permeability and conductivity. The increase in conductivity can cause lossy medium, where the radio waves can be significantly attenuated. Therefore, the EM wave attenuates with the increase in the complex propagation constant, as specified in Equation (4).
Another major issue with tissue diversification is that the radio wave propagation speed decreases because of the complex inhomogeneous characteristics of biological tissue. Therefore, the radio wave propagation speed primarily depends on the permittivity and conductivity of the medium. Additionally, the propagation speed in any medium is characterized based on phase ( V p ) and group ( V g ) velocity as [ 79 , 82 ]
where β is the phase constant, defined from the complex propagation constant as [ 83 ]
In Equations (9) and (10), the propagation speed is characterized based on the phase constant, and it decreases with the increase in the conductivity of the medium. In contrast, the rise in the frequency influences the increase in propagation speed. In summary, higher conductivity of biological tissues can cause significant reduction in the propagation speed of radio waves.
4.2. Impact of Effective Wavelength on In-Body Antenna
In Ref. [ 79 ], the effective wavelength λ in any medium is defined as
The phase constant β is dependent on conductivity σ proportionally. The rise in medium conductivity reduces λ, which therefore leads to miniaturization of the in-body antenna. In an ideal case, in order to facilitate a surgical procedure, the IBBDs have to be in the range of 1 to 10 mm in diameter for a length of 5 to 35 mm, while in the MedRadio and ISM bands, the free-space wavelength is approximately 74 and 12 cm, respectively [ 78 ]. This indicates that in-body antennas must be profoundly miniaturized, leading to antenna dimensions of some fractions of the free-space wavelength (typically λ /30 and λ /5 for the MedRadio and ISM bands, respectively).
4.3. Effect of Efficiency
In a free space, the radiated power of an antenna depends on the far field elements only, as the near field is mostly reactive and therefore not distressing the radiated or the absorbed power [ 78 ]. In the case of an antenna radiating into lossy matter, the near field component plays a key role by causing strong coupling with the surrounding medium near to the antenna and hence increases the losses. Therefore, the total radiated power primarily depends on the radial distance r . The outer boundaries of the near field and far field are commonly assumed as r < 0.62 D 3 / λ and r < D 2 / λ , where D is the antenna’s highest dimension [ 84 ]. In the case of in-body antennas, the situation improves slightly because the complex lossy medium surrounding the antenna is of finite dimensions. In the lossy medium, strong coupling with nearby lossy biological tissues is caused by the radiated radio wave. Therefore, the coupling of frequency causes a loss of radiated power. This coupling is also the primary driver of lower radiation efficiency of in-body antennas. Furthermore, biocompatibility encapsulation using insulating materials plays a key role in reducing the coupling with the adjacent lossy environment [ 78 ].
4.4. Biocompatible Encapsulation
The process of covering the in-body antenna with biocompatible material is known as encapsulation. In Ref. [ 78 ], the effect of encapsulation on radiation efficiency is described through a comparative study between Zirconia and PEEK used as encapsulation shells for in-body antennas. It was observed that Zirconia demonstrates better results than PEEK due to its significantly lower loss and higher dielectric constant, which agrees with a higher concentration of the near field in the low-loss surrounding of the in-body antenna. Furthermore, a thicker encapsulation facilitates overall low losses. However, in the case of PEEK, this effect reaches saturation after a thickness of 2 mm, where the losses are approximately similar for a thickness of 3 mm. It was also noticed that PEEK is the kind of material, which can be handled and manufactured far more easily than Zirconia, thus being more suitable for IBBDs. In summary, low-loss encapsulation helps mitigate the loss by concentrating the near field in a low-loss region.
4.5. Effect on Antenna Bandwidth
In-body antennas are compact in size and subject to narrower bandwidth [ 78 ]. However, all the radiated power from a transmitter does not reach the receiver because of significant absorption and reflection by the biological tissue medium. In an in-body antenna, the absorbed power is commonly greater than the reflected power, which generally causes the bandwidth to be wider. This also causes lower radiation efficiency of the antenna. It is possible to reduce these losses by using bio-encapsulation—as discussed in the previous section—and impedance matching, making the bandwidth narrower. However, an in-body antenna with narrow bandwidth suffers from frequency detuning inside the biological tissue environment. Therefore, a cautious consideration is necessary to solve this issue. The bandwidth of the in-body antenna can be improved by using a thicker substrate. In Ref. [ 85 ], the bandwidth of an implantable monopole antenna is improved by connecting a strip line with U-shaped ground. In Ref. [ 86 ], the ground plane of the implantable PIFA antenna is partly connected to a RFID circuit to enhance the bandwidth.
4.6. Effect on Antenna Radiation Pattern
The lossy medium present in a human body environment can cause broadening of the radiation pattern because of the reflection, refraction and scattering existing in or generated from the body tissues [ 78 ]. The radiation pattern of an in-body antenna would also be variable in the same medium if the mounting circumstances and in-body positions were different.
4.7. SAR Requirement
As explained in Section 2.6 , SAR is used as a metric to guarantee the safety of biological tissues in the event of severe electromagnetic exposure. The standard SAR levels are maintained by IBBDs by considering low output power. In general, the specific absorption ( SA ) per pulse can be calculated by [ 87 ]
where T p represents the pulse duration. The EM power absorbed by the biological tissue medium can raise the temperature of the tissue. It must be noted that the temperature of the biological tissues adjacent to the implanted device should not rise more than 1–2 °C.
4.8. Effective Isotropic Radiated Power (EIRP)
A remarkable level of EIRP of the in-body antenna can be harmful to the biological tissues, and it can create interference with the nearby radio devices. The standardized limit of EIRP for an in-body antenna functioning in the MedRadio band is −16 dBm [ 88 ] and −36 dBm at 915 MHz for the ISM band [ 89 ]. In case the in-body antenna is used for data telemetry, the input power must be limited to alleviate damage to the tissues. If the in-body antenna is operating as a receiver, the external source of power must follow the aforementioned standards.
4.9. Powering
Continuous power delivery to the IBBDs is one of the foremost challenges for in-body antennas. The current battery technologies are an inefficient solution for such application due to their short lifetime [ 11 , 14 ]. Furthermore, batteries contain hazardous ingredients and necessitate a surgical operation to be replaced. Additionally, the power system of IBBDs must be lightweight and easy to fabricate to facilitate an easy movement of patients. It is also necessary to maintain the energy level of the system in the design of a powering system for IBBDs.
5. In-Body Antenna Applications
This section explains the range of applications of IBBDs (implantable, ingestible and injectable devices) implemented with different types of in-body antennas.
5.1. Pacemaker
A compact meander line planar implantable antenna for a pacemaker application operating at 402.5 MHz with a bandwidth of 33.5% is presented by Samsuri et al. in Ref. [ 90 ]. The proposed antenna is implemented on a FR-4 substrate with ε r = 4.7 and tan δ = 0.025 with a size of 30.5 mm × 21.02 mm × 6.4 mm, as shown in Figure 9 . The antenna performance is evaluated through simulation in a multi-layer human body model.

Compact meander line planar implantable antenna for a pacemaker [ 90 ]. Reproduced with permission from an open-access article from the Indonesian Journal of Electrical Engineering and Computer Science (CC-BY).
Figure 10 shows a tiny and compact implantable planar antenna with the size of 3 mm × 3 mm × 0.5 mm as presented in Ref. [ 25 ] for a wireless cardiac pacemaker. Rogers 3010 is used as a superstrate and substrate where ε r = 10.2 and tan δ = 0.0023. The antenna is optimized and loaded with a defective slotted structure to improve the efficiency and overall performance of the antenna in an ISM frequency band of 2.4 to 2.48 GHz. The definite bandwidth of the antenna is 22%, with the peak gain of −24.9 dBi.

Tiny and compact implantable planar antenna for a wireless cardiac pacemaker [ 25 ]. Reproduced with permission from an open-access article from Scientific Reports (CC-BY).
A triband spiral shaped implantable antenna is presented by Shah et al. in Ref. [ 68 ] with slotted ground operating at 402 MHz, 1.6 GHz and 2.45 GHz for a leadless pacemaker system. The size of the antenna is 7 mm × 6.5 mm × 0.377 mm, where Rogers RT/Duroid 6010 with ε r = 10.2 and tan δ = 0.0035 is utilized as a superstrate and substrate, as shown in Figure 11 . The gains of the antenna at the three different frequencies are −30.5 dBi, −22.6 dBi, −18.2 dBi, respectively, with bandwidths of 36.8%, 10.8% and 3.4%, respectively.

Triband spiral shaped antenna and its test setup in minced pork [ 68 ]. Copyright © IEEE .
5.2. Blood Pressure Monitoring Implant
The frequent rise and drop in blood pressure can originate a stroke or severe cardiovascular disease for patients, which necessitates the requirement for an accurate blood pressure monitoring system in a healthcare space.
The measurement of blood pressure through an implantable antenna system embedded into the heart would be an outstanding solution for risky heart patients. In Ref. [ 23 ], a pseudo-normal-mode helical antenna is presented with a poly-siloxane (PDMS) insulation layer, as shown in Figure 12 . The operation frequency of the antenna is 863–870 MHz with a size of 3 mm in diameter and 9.44 mm in height. The antenna is built with a 0.33 mm diameter nitinol wire. The implant antenna and sensor are put inside the left ventricle and subjected to experimentation with a pig, as shown in Figure 8 . This antenna can provide a maximum radiation efficiency of −27 dB and directivity of 2.65 dBi.

Helical wire antenna for blood pressure monitoring implant [ 23 ]. Reproduced with permission from an open-access article from Biomedical Microdevices (CC-BY).
A smart stent antenna is presented in Ref. [ 91 ] for intravascular monitoring, as shown in Figure 13 . The commonly used L-605 Cobalt–Chromium (Co–Cr) alloy is used as the material for the stent. The diameter and length of the stent are 2 mm and 18 mm, respectively. This antenna can achieve a gain of 1.38 dBi and a radiation efficiency of 74.5% at a resonant frequency of 2.07 GHz.

Smart stent antenna for intravascular monitoring [ 91 ]. Reproduced with permission from an open-access article from MDPI Sensors (CC-BY).
5.3. Brain Implant
Figure 14 shows a miniaturized planar implantable antenna presented in Ref. [ 92 ] with an operation frequency of 2.4 GHz. The approximate size of the antenna is 10 mm × 10 mm × 1.5 mm, and it is manufactured with Taconic RF-35 with ε r = 3.5 and tan δ = 0.0018. The achieved bandwidth of the antenna is 14.9%, with the peak gain of −20.75 dBi.

Miniaturized planar implantable antenna for a brain implant [ 92 ]. Reproduced with permission from an open-access article from IEEE Access (CC-BY).
In Ref. [ 93 ], a 2.4 or 4.8 GHz planar implantable antenna with modified E-shape is presented, built with a Rogers TMM13i substrate with ε r = 12.2 and tan δ = 0.0019. The size of the implantable antenna is 10 mm × 8.7 mm × 0.76 mm. The maximum SAR achieved is 69 mW/Kg for 10 g of tissue.
The antenna presented in Ref. [ 68 ] is also compatible with a pacemaker application for a brain implant.
A novel flexible moon-shaped slot implantable antenna operating at 2.45 GHz frequency is presented for neural recording systems and brain implants in Ref. [ 94 ]. The size of the antenna is 8 mm × 9 mm × 0.2 mm, fabricated with a RO4003C substrate with ε r = 3.48 and tan δ = 0.0027. The peak gain achieved is approximately −13 dBi at 2.45 GHz. The maximum SAR achieved is less than 1 W/kg for 1 g of tissue.
5.4. Intracranial Pressure
Figure 15 shows a miniaturized planar implantable antenna proposed by Shah et al. for intracranial pressure monitoring at 915 MHz and 2.45 GHz [ 95 ]. The proposed antenna has a size of 8 mm × 6 mm × 0.5 mm and utilizes Rogers 6010 as the substrate with ε r = 10.2 and tan δ = 0.0023. Biocompatibility is confirmed by the ceramic alumina encapsulation. The antenna achieved a gain and bandwidth of −28.5 dBi and 9.84% at 915 MHz, respectively, and −22.8 dBi and 8.57% at 2.45 GHz, respectively. To achieve the safety limit of 2 W/kg for SAR 10g , the maximum allowable input power is 17.12 mW at 915 MHz and 20.6 mW at 2.45 GHz.

Miniaturized planar implantable antenna for intracranial pressure [ 95 ]. Copyright © IEEE .
A coplanar miniature antenna with the size of 6 mm × 5 mm × 1 mm is presented in Ref. [ 96 ] with an operation frequency of 2.45 GHz, as shown in Figure 16 . Khan et al. claimed to use low-permittivity polyimide as a flexible substrate for the proposed antenna. This antenna can provide a peak gain of −19.63 dBi and maximum SAR of 10 mW/kg in brain tissue.

Coplanar miniature antenna for intracranial pressure tested in liquid phantom [ 96 ]. Reproduced with permission from an open-access article from the International Journal of Antennas and Propagation (CC-BY).
In Ref. [ 97 ], a PIFA antenna design technique was explained for head-implanted medical devices, including intracranial pressure application. In this work, Rogers RO 3210 is selected as the substrate with ε r = 10.2 and tan δ = 0.003. The antenna is 12 mm in diameter and 1.8 mm in width. It was designed to operate at 402, 433, 868 and 915 MHz. It can achieve a SAR of 2 W/kg for 10 g of tissue when the input power is 4.927 mW. It can achieve a peak gain of −36.90, −35.99, −35.14 and −32.94 dB at 402, 433, 868 and 915 MHz, respectively.
A wireless power receiver spiral antenna with dimensions of 12.88 mm × 13.46 mm × 0.05 mm is presented by Waqas et al. in Ref. [ 98 ] for an intracranial pressure implant. The antenna is made on a flexible polyimide substrate with ε r = 3.3 and tan δ = 0.002. The operating frequency of the antenna was selected as 11 MHz, where a −2.17 dB measurement peak gain was achieved. The wireless power transfer efficiency was 1.18%.
5.5. Glucose Monitoring and Sensing
In Ref. [ 99 ], a miniaturized single-fed wide-beamwidth circularly polarized implantable antenna working in the ISM band (2.40–2.48 GHz) is presented for subcutaneous real-time glucose level monitoring application. Figure 17 shows the proposed antenna with the dimensions of 8.5 mm × 8.5 mm × 1.27 mm employing four C-shaped slots and a complementary split-ring resonator (CSRR). Meanwhile, by adjusting the slits of the CSRR, circular polarization is realized. In this work, Rogers 3210 is selected as the substrate with ε r = 10.2 and tan δ = 0.003. The simulation results with a three-layer phantom demonstrate that the impedance bandwidth is 12.2%, with a peak gain of −17 dBi.

Proposed wide-beamwidth circularly polarized implantable antenna for glucose monitoring presented in Ref. [ 99 ]. Copyright © IEEE .
Mujeeb-U-Rahman et al. in Ref. [ 100 ] presented a spiral antenna for wireless power and data telemetry for an injectable glucose sensing device. The size of the antenna is 3 mm × 0.6 mm. It is operating at the 900 MHz ISM band, with the peak power gain of less than −30 dB. The power transfer efficiency of the inductive link is 0.1%. The antenna is built on a silicon substrate through a photolithography process, as shown in Figure 18 .

Injectable glucose sensing device with spiral antenna [ 100 ]. Reproduced with permission from an open-access article from Scientific Reports (CC-BY).
5.6. Orthopedic Implant Infection Monitoring
A planar implantable antenna for monitoring infection in an orthopedic implant is presented in Ref. [ 101 ] for the 860 to 960 MHz RFID ultra-high frequency (UHF) band, as shown in Figure 19 . The size of the antenna is 14 mm × 6 mm × 3 mm. It is fabricated on a FR4 substrate and achieves a peak gain of −22 dBi.

Planar implantable antenna for an UHF RFID-based orthopedic implant [ 101 ]. Copyright © IEEE .
5.7. Cochlear Implant
A folded loop antenna built with a metal wire with the size of 38 mm × 38 mm × 2.2 mm and a wire radius of 0.3 mm is presented in Ref. [ 102 ] for a cochlear implant, as shown in Figure 20 . The operating frequency of the proposed antenna is 2.45 GHz, with a bandwidth of 8.57%. It realizes a gain of −0.1 dBi.

Folded loop wire antenna for a cochlear implant [ 102 ]. Reproduced with permission from Attribution-NoDerivaties 4.0 International shared at CSEM archive.
5.8. Retinal Implant
A PIFA antenna with a MedRadio (401–406 MHz) band is presented by Orfeas and Nikita in Ref. [ 103 ] for a retinal implant. The antenna diameter is approximately 12 mm with a thickness of 1.8 mm in PEEK encapsulation. In this work, Rogers 3210 is selected as the substrate with ε r = 10.2 and tan δ = 0.003. This antenna achieves a peak gain of −36.82 dBi with a bandwidth of 3.4% in PEEK encapsulation. It can achieve a SAR of 2 W/kg for 10 g of tissue when the input power is 21 mW in PEEK encapsulation.
5.9. Capsule Endoscopy
Capsule endoscopy (CE) is the most common ingestible device, which is used for diagnosis and monitoring of different gastrointestinal (GI) disorders. A wide range of in-body antennas used for CE application over the last few years are described below.
A planar meandering antenna fabricated on a 0.1 mm thick polyimide flexible substrate (with ε r = 3.5 and tan δ = 0.0027) with 0.035 mm copper thickness is presented in Ref. [ 104 ], as shown in Figure 21 . The proposed antenna has dimensions of 28 mm × 12 mm, working with an operation frequency of 433 MHz. The measured peak gain is −39 dBi.

Fabricated planar meandering antenna with SMA connection [ 104 ]. Reproduced with permission from an open-access article from IEEE Access (CC-BY).
In Ref. [ 105 ], a circularly polarized (CP) helical implantable antenna is proposed. The proposed antenna operates at 2.4 GHz frequency with a gain of −19.83 dBi and a bandwidth of 290 MHz. A perfect electric conductor (PEC) is used to build and simulate this antenna with a 6.6 mm diameter and 8.85 mm length, with an approximate wire thickness of 0.4 mm. Furthermore, the antenna covers the cylindrical shape of the capsule with a diameter of 7.06 mm and length of 25 mm.
A 3D wireless power transfer receiver coil of 8.9 mm in diameter and 4.8 mm in thickness is presented in Ref. [ 61 ], as shown in Figure 22 . The proposed 3D coil is built with a 0.2 mm copper wire. It achieves 0.7% power transfer efficiency (PTE) and a SAR of 66 mW/kg for 10 g of tissue at 1 MHz operation frequency.

Three-dimensional wireless power transfer receiver coil for capsule endoscopy tested in gel phantom [ 61 ]. Reproduced with permission from an open-access article from MDPI Micromachines (CC-BY).
A conformal antenna with a frequency of 402, 433, 915 and 2450 MHz is presented with an achieved gain of −32.5, −30.4, −17.9 and −19.0 dBi, respectively [ 106 ]. The proposed antenna is printed on a 0.17 mm thick flexible Kapton substrate with ε r = 3.5 and tan δ = 0.0027. It has a size of 12 mm × 6 mm. The bandwidth of the antenna is reported to be 2.95 and 3.33 GHz.
A similar conformal differentially fed antenna with an operation frequency of 915 MHz is presented in Ref. [ 107 ]. The proposed antenna has dimensions of 32 mm × 5.8 mm, as shown in Figure 23 , and it is printed on a flexible polyimide substrate (with ε r = 3.5 and tan δ = 0.008) of 0.15 mm in thickness. The antenna achieves a peak gain of −21 dBi and a bandwidth of 8.9% tested in the phantom under 50 mm depth.

Conformal differentially fed antenna built in a capsule [ 107 ]. Copyright © IEEE .
Figure 24 shows a slot line fed antenna with the size of 7 mm × 7 mm constructed on a silicon substrate of approximately 0.675 mm in thickness with ε r = 11.9, which is presented in Ref. [ 108 ]. The proposed antenna is operating at a 915 MHz frequency. It can achieve a peak gain of −35.5 dBi and a bandwidth of 300 MHz, as tested in colon phantom. Furthermore, it achieves a SAR of 8 mW/kg for 1 g of colon tissue.

Miniaturized slot line fed antenna for capsule endoscopy [ 108 ]. Reproduced with permission from an open-access article from IET Microwaves, Antennas & Propagation (CC-BY).
A wideband multiple-input–multiple-output (MIMO) compact antenna for ingestible capsules is presented in Ref. [ 109 ], as shown in Figure 25 . The size of the antenna is 5 mm × 4.2 mm × 0.12 mm, operating at a 2.45 GHz frequency. The proposed MIMO antenna is manufactured on a Rogers RO3010 substrate with ε r = 10.2 and tan δ = 0.0022. It maintains a peak gain of −20.6 dBi and a bandwidth of 25%. It can achieve a SAR of 2 W/kg for 10 g of tissue when the input power is 3.97 mW.

Fabricated MIMO antenna tested inside minced meat [ 109 ]. Reproduced with permission from an open-access article from Scientific Reports (CC-BY).
5.10. Cell Rover
In Ref. [ 110 ], a sub-micrometer-sized injectable wire antenna is presented for the purpose of smart sensing, modulation, as well as energy harvesting to power in-cell nano-electronic computing. Figure 26 shows the proposed cell rover device, which can help understand the cell biology for different diagnostic and therapeutic applications. The receiver coil antenna is built with an American Wire Gauge (AWG) 47 wire, with a thickness of 0.0355 mm. The diameter and length of the coil are 2 mm and 1 mm, respectively. The proposed antenna operates at a 4.5 MHz frequency. It can achieve a PTE of 0.63% and a SAR of 0.0226 mW/kg for 10 g of tissue.

Cell rover with wire antenna inserted into the cell membrane [ 110 ]. Reproduced with permission from an open-access article from Nature Communications (CC-BY).
5.11. Pharmacology and Optogenetics
In Ref. [ 111 ], an injectable IBBD is presented for pharmacology and optogenetics applications, as shown in Figure 27 . Pharmacology and optogenetics are widely used in neuroscience research to study the central and peripheral nervous systems. This IBBD includes a spiral antenna of approximately 5 mm in diameter, operating at a 13.56 MHz frequency. The spiral antenna is fabricated on a flexible sheet of copper clad polyimide.

Wireless, battery-free, injectable microsystems for programmable pharmacology and optogenetics applications [ 111 ]. Reproduced with permission from an open-access article from PNAS-Biological Sciences (CC-BY).
Table 1 , Table 2 and Table 3 summarize the different types of in-body antennas for implantable, ingestible and injectable IBBD applications, respectively, discussed in this section, highlighting their structure (type and substrate) and key performance parameters (size, gain, −10 dB bandwidth and SAR).
Summary of different types of in-body antennas used in implantable IBBD applications.
Summary of different types of in-body antennas used in ingestible IBBD applications.
Summary of different types of in-body antennas used in injectable IBBD applications.
6. Some Future Research Challenges
This section describes some of the limitations of the current in-body antenna designs and future challenges, which need to be resolved to improve performance.
- Generally, the coupling of in-body antennas with lossy tissue causes the absorption of the EM wave in the near reactive and far field, which is commonly not considered in the design phase. This results in a significant reduction in the radiation efficiency and peak gain, causing inefficient antenna operation. This is inevitable in the far field. However, it is possible to reduce the absorption of the EM wave by covering the in-body antenna with biocompatible material in the near field. Therefore, designing in-body antennas with biocompatibility covering the near field will be a conceivable future research challenge.
- The human body is formed with inhomogeneous biological tissues and organs. Furthermore, the characteristics and dimensions of biological tissues vary every so often, including by gender. Therefore, the detuning effect of the in-body antenna inside the human body is considered as one of the primary research and design IBBD applications. To date, in-body antenna design and experiments are mostly restricted only to a single tissue environment, which will be a noteworthy shortcoming for diverse biological tissue environments. Therefore, the upcoming in-body antenna research focus must be the investigation of diverse biological environments for efficient in-body antenna operation.
- The implantation of a device operating at radio frequency inside the biological tissue may lead to a severe long-term health problem due to radiative power absorption. Therefore, an effective and optimized in-body antenna design with a SAR value limit as standard and an appropriate selection of biocompatible materials will be the key future research investigation.
- Traditional antenna miniaturization techniques tend toward narrow operational bandwidths. Such narrowband operation can cause a detuning effect of the in-body antenna inside the biological environment. Biocompatible encapsulation of the in-body antenna can be utilized to increase the radiation efficiency and gain. However, this inflates the overall IBBD thickness. Therefore, an antenna design technique with acceptable operational bandwidth, radiation efficiency and gain is still a challenging matter in IBBDs. Increasing the operation frequency band can increase the miniaturization scale. However, this increases the loss and tissue absorption, which introduces additional design challenge.
- Lastly, battery-powered IBBDs have a limited lifetime and bulky dimension, which can cause insufficiency in an in-body antenna power system. Furthermore, the replacement of the battery through a surgical procedure is complex and costly. Therefore, designing power-efficient IBBDs for in-body antennas is a crucial design challenge for the future.
7. Conclusions
A comprehensive review is presented for in-body antennas for IBBDs (implantables, ingestibles, injectables). Designing an in-body antenna operating in a harsh bio-tissue environment is a challenging task, where several specifications are required to be considered, including the operation frequency, band selection, size, performance (gain, efficiency, radiation pattern), biocompatibility and patient safety. Widely used miniaturization techniques, such as high-permittivity substrates, lengthier current flow path on the radiator, inductive or capacitive loading, pin shorting, higher operating frequencies, ground plane modifications and use of metamaterials, are discussed, and each technique is evaluated in terms of its merits and issues. This study shows that despite having the superior potential of miniaturization, higher frequency usage suffers from significant losses, which requires additional investigations in this domain, as radio wave exposure studies and research works at these frequencies are not yet well established. Moreover, this paper reviews the antenna design and manufacturing process and illustrates the antenna testing procedures, including in vitro and in vivo testing. This paper also summarizes several existing in-body antenna designs, including planar, PIFA, wire, conformal, spiral, slotted and MIMO structures, and classifies antennas according to the IBBD applications. Although the selection of an antenna type depends on specific applications, patch-type planar structures have been more commonly adapted by researchers, as shown in Table 1 . The study of patient safety is one of the primary requirements for IBBDs, which is strictly administered through the SAR and EIRP limits. Table 1 shows that most researchers ignore this step while designing in-body antennas for IBBDs, which is alarming, as it fails to demonstrate the suitability of the proposed antennas for in-body applications. In-body antennas are obligated to meet these regulations while considering the bio-tissue environment as the key testing platform and further confirming the safety profile through utilization of biocompatible materials. Finally, the article concludes with listing some of the drawbacks and limitations of existing in-body antenna technology and the forthcoming research challenges in this area.
Funding Statement
This research is funded by Heriot-Watt University Kickstart Research fund granted to Sadeque Reza Khan.
Author Contributions
Conceptualization, I.N. and S.R.K.; methodology, S.R.K.; software, I.N. and S.R.K.; validation, K.A. and I.N.; resources, K.A. and S.R.K.; writing—original draft preparation, S.R.K.; writing—review and editing, K.A., I.N. and S.R.K.; supervision, S.R.K.; funding acquisition, K.A. and S.R.K. All authors have read and agreed to the published version of the manuscript.
Data Availability Statement
Conflicts of interest.
The authors declare no conflict of interest.
Disclaimer/Publisher’s Note: The statements, opinions and data contained in all publications are solely those of the individual author(s) and contributor(s) and not of MDPI and/or the editor(s). MDPI and/or the editor(s) disclaim responsibility for any injury to people or property resulting from any ideas, methods, instructions or products referred to in the content.
Design, Analysis and Applications of Wearable Antennas: A Review
Ieee account.
- Change Username/Password
- Update Address
Purchase Details
- Payment Options
- Order History
- View Purchased Documents
Profile Information
- Communications Preferences
- Profession and Education
- Technical Interests
- US & Canada: +1 800 678 4333
- Worldwide: +1 732 981 0060
- Contact & Support
- About IEEE Xplore
- Accessibility
- Terms of Use
- Nondiscrimination Policy
- Privacy & Opting Out of Cookies
A not-for-profit organization, IEEE is the world's largest technical professional organization dedicated to advancing technology for the benefit of humanity. © Copyright 2024 IEEE - All rights reserved. Use of this web site signifies your agreement to the terms and conditions.

IMAGES
VIDEO
COMMENTS
The paper presents the. design analysis of rectangular and square shaped microstrip. an tenna. Both the antennas used microstrip line for feeding. purpose. The square-shaped microstrip antenna is ...
Conclusion In this work, a microstrip patch slot antenna for 5G and satellite communication has been designed. The simulation of the design has been carried out in Ansys HFSS v.15.0 simulation tool. The obtained results are found to be satisfying the requirements of the 5G communication antenna. The antenna works at a resonating frequency of 43 ...
In our implementation, the algorithm converged after 12 iterations giving as result an evolved patch antenna with a footprint of 16.88 × 13.76 mm. 2. resonating at around 3.89 GHz with an S. 11 ...
This paper provides a comprehensive study of different antenna designs considering various 5G antenna design aspects like compactness, efficiency, isolation, etc. This review paper elaborates the state-of-the-art research on the different types of antennas with their performance enhancement techniques for 5G technology in recent years.
This work presents an optically transparent and flexible MIMO antenna that features two square patch elements placed in close proximity, aiming to meet the demands of compactness, flexibility ...
This paper primarily focuses on well-organized information about MIMO antenna design approaches with specific methodologies in order to achieve the desired antenna performance. Moreover, the comparison tables provided in the manuscript will help readers to implement and modify the described techniques for better performance of MIMO antennas.
In this manuscript a compact multiband antenna with dimension 16 × 18 × 0.787 mm3 is presented for multiple wireless applications including Digital Cellular System (1.71-1.88 GHz), Personal Communication System (1.85-1.99Gz), Bluetooth Wireless System (2.402-2.480 GHz), WiMAX (3.30-3.80 GHz), WLAN (5.150-5.825 GHz) and X-Band Downlink System (7.25-7.75 GHz). Radiating patch ...
The primary goal of this paper is to discuss the spectrum used or expected to be used for 5G communication, the required specifications of 5G antennas, the various issues, challenges, and research gaps in 5G antenna design, a review of popular antenna design technologies used in 5G, and a comparative analysis of some potential antennas used for ...
This paper discusses various challenges related to the antenna design process for 5G communications focusing on the 26 GHz band. In a first example, an 8 element wideband array antenna is optimized for use in a mobile phone. The antenna performance is investigated, including gain, coupling and beamsteering. Aspects related to the antenna integration workflows are also discussed. In a second ...
The antenna design substrate is FR4 (lossy) with a dielectric constant (Er) of 4.3 dielectric material, and the ground and patch materials are copper (annealed). The substrate is 71.62mm in width and 55.47mm in length. The height of the dielectric material is 1.6mm, which is the normal size for FR4 material. The conducting patch element has a ...
In this age of information technology, wireless communication is becoming more popular every day. And these wireless applications have some effect on the antenna. In this paper, a microstrip patch antenna design, simulation, and analysis are done. And to make this design, Rogger RT/Duroid 5880, whose dielectric permittivity is 4.3, has been used as the substrate material. Besides, antenna ...
In this paper, in order to find a suitable metallic liquid for effective antennas, we design and compare the performance of metallic liquid antennas using Mercury, gallium indium alloy (EGaIn ...
This paper proposes a new antenna design that is able to increase the performance level of IoT applications by means of an original design. The antenna was designed, simulated, tested, and evaluated in a real operating scenario. ... In recent years, the research interest in wearable antennas inserted on flexible materials [15,16,17] has ...
14 Mar 2024. 13 Mar 2024. International Journal of Antennas and Propagation publishes research on the design, analysis, and applications of antennas, along with studies related to the propagation of electromagnetic waves through space, air, and other media.
Radio Science is an AGU journal publishing original research articles on radio frequency electromagnetic-propagation and its applications. Abstract This paper presents the review on the design of various reconfigurable antennas and their applications in the current scenario. ... There are more challenges involved in the design of these antennas ...
Abstract. The Research into microstrip patch antenna has made great strides in recent years. Compared to conventional microstrip patch, microstrip patch horns have the advantages of being low-cost, which is why they can be built in bulk, easily integrated with integrated microwave circuits (MICs), dual and triple, Low cost, Low size, Low weight, High Performance, and low volume.
Feature papers represent the most advanced research with significant potential for high impact in the field. A Feature Paper should be a substantial original Article that involves several techniques or approaches, provides an outlook for future research directions and describes possible research applications. ... The antenna design is explained ...
The antenna is a critical part of the RF front end of a communication system. In this study, we present some of the major applications of artificial intelligence (AI) to antenna design. We review the previous research and applications of several AI techniques such as evolutionary algorithms, machine learning, and knowledge representation ontologies. Applications may vary from antenna design to ...
The main challenges in these proposed antennas are volume and occupied areas. Therefore, this paper aims to design, simulate, and analyze an antenna with a reduced volume destined for GPS application. The rest of this paper is organized as follows: In section 2, the antenna design steps are presented. The results and discussion are given in ...
This review work aims to describe the design specifications, implementation and testing techniques, challenges and different applications of in-body antennas. Following the Introduction, the paper is divided into six sections. Section 2 briefly highlights the design specifications of in-body antennas.
This paper discusses the state-of-the-art wearable/textile/flexible antennas integrated with metamaterial structures composed of wearable/flexible substrate materials, with a focus on single and dual band antenna designs. The paper also reviews the critical design issues, various fabrication techniques, and other factors that need to be ...