Cultivating a Greener Tomorrow: Sustainable Agriculture Strategies for Minimizing Agricultural Waste
- First Online: 15 March 2024

Cite this chapter
- Dipti Bharti 4 ,
- Abhilekha Sharma 5 ,
- Meenakshi Sharma 6 ,
- Rahul Singh 4 ,
- Amit Kumar 7 &
- Richa Saxena 8
71 Accesses
Agriculture that is truly sustainable must incorporate social, economic, and environmental sustainability. Increased awareness of the need for enhanced agricultural productivity stems from the requirement to feed a growing population. It is interesting that virtually all agricultural activities generate waste, and many nations produce substantial volumes of waste. Recent observations from our study reveal that globally, agricultural activities generate approximately five billion tons of waste each year. However, the improper disposal of these wastes can lead to staggering financial losses, estimated at around $300 billion annually, and severe environmental contamination, posing significant threats to human health. Every state and nation, on average, produces a substantial amount of waste every year, estimated at 200 million tons, or every 6 months. Hence, it is imperative that this waste is disposed of properly, recycled, or utilized to create value for the environment and agriculture. We find that “reducing,” “reusing,” and “recycling” field waste can significantly reduce the environmental footprint of agricultural practices, potentially reducing greenhouse gas emissions by up to 25% and conserving water resources by 15%. This chapter focuses on effective strategies for managing these wastes, including building a more sustainable agricultural supply chain, developing long-lasting markets, and enhancing rural infrastructures such as roads, storage, and power.
This is a preview of subscription content, log in via an institution to check access.
Access this chapter
- Available as PDF
- Read on any device
- Instant download
- Own it forever
- Available as EPUB and PDF
- Durable hardcover edition
- Dispatched in 3 to 5 business days
- Free shipping worldwide - see info
Tax calculation will be finalised at checkout
Purchases are for personal use only
Institutional subscriptions
Agamuthu, P. (2009). Challenges and opportunities in agro-waste management: An Asian perspective. In Inaugural meeting of first regional 3R forum in Asia (Vol. 11, 12). University of Malaysia.
Google Scholar
Altieri, M. A. (2002). Agroecology: The science of natural resource management for poor farmers in marginal environments. Agriculture, Ecosystems & Environment, 93 (1–3), 1–24.
Article Google Scholar
Benatti, A. L. T., & Polizeli, M. D. L. T. D. M. (2023). Lignocellulolytic biocatalysts: The main players involved in multiple biotechnological processes for biomass valorization. Microorganisms, 11 (1), 162.
Article CAS Google Scholar
Blay-Palmer, A., Sonnino, R., & Custot, J. (2016). A food politics of the possible? Growing sustainable food systems through networks of knowledge. Agriculture and Human Values, 33 , 27–43.
Bosecker, K. (1997). Bioleaching: Metal solubilization by microorganisms. FEMS Microbiology Reviews, 20 (3–4), 591–604.
Boserup, E. (1975). The impact of population growth on agricultural output. The Quarterly Journal of Economics, 89 (2), 257–270.
Bouallagui, H., Touhami, Y., Cheikh, R. B., & Hamdi, M. (2005). Bioreactor performance in anaerobic digestion of fruit and vegetable wastes. Process Biochemistry, 40 (3–4), 989–995.
Bracco, S., Calicioglu, O., Gomez San Juan, M., & Flammini, A. (2018). Assessing the contribution of bioeconomy to the total economy: A review of national frameworks. Sustainability, 10 (6), 1698.
Brennan, L., & Owende, P. (2010). Biofuels from microalgae—A review of technologies for production, processing, and extractions of biofuels and co-products. Renewable and Sustainable Energy Reviews, 14 (2), 557–577.
Chi, X., Wang, M. Y., & Reuter, M. A. (2014). E-waste collection channels and household recycling behaviors in Taizhou of China. Journal of Cleaner Production, 80 , 87–95.
Chiellini, E., Corti, A., & Swift, G. (2003). Biodegradation of thermally-oxidized, fragmented low-density polyethylenes. Polymer Degradation and Stability, 81 (2), 341–351.
Conti, F., Toor, S. S., Pedersen, T. H., Seehar, T. H., Nielsen, A. H., & Rosendahl, L. A. (2020). Valorization of animal and human wastes through hydrothermal liquefaction for biocrude production and simultaneous recovery of nutrients. Energy Conversion and Management, 216 , 112925.
Converti, A., Casazza, A. A., Ortiz, E. Y., Perego, P., & Del Borghi, M. (2009). Effect of temperature and nitrogen concentration on the growth and lipid content of Nannochloropsis oculata and Chlorella vulgaris for biodiesel production. Chemical Engineering and Processing: Process Intensification, 48 (6), 1146–1151.
Cordell, D., Drangert, J. O., & White, S. (2009). The story of phosphorus: Global food security and food for thought. Global Environmental Change, 19 (2), 292–305.
Diaz, L. F., Savage, G. M., & Eggerth, L. L. (2005). Alternatives for the treatment and disposal of healthcare wastes in developing countries. Waste Management, 25 (6), 626–637.
Diener, S., Zurbrügg, C., & Tockner, K. (2009). Conversion of organic material by black soldier fly larvae: Establishing optimal feeding rates. Waste Management & Research, 27 (6), 603–610.
Dorward, A., Poole, N., Morrison, J., Kydd, J., & Urey, I. (2003). Markets, institutions and technology: Missing links in livelihoods analysis. Development Policy Review, 21 (3), 319–332.
Duque-Acevedo, M., Belmonte-Ureña, L. J., Cortés-García, F. J., & Camacho-Ferre, F. (2020). Agricultural waste: Review of the evolution, approaches and perspectives on alternative uses. Global Ecology and Conservation, 22 , e00902.
Erb, K. H., Lauk, C., Kastner, T., Mayer, A., Theurl, M. C., & Haberl, H. (2016). Exploring the biophysical option space for feeding the world without deforestation. Nature Communications, 7 (1), 11382.
Ezekannagha, E. (2020). Assessing the climatic suitability of Bambara groundnut as an underutilised crop to future climate projections in Sikasso and Ségou, Mali (Master’s thesis, Faculty of Science).
Godfray, H. C. J., Beddington, J. R., Crute, I. R., Haddad, L., Lawrence, D., Muir, J. F., Pretty, J., Robinson, S., Thomas, S. M., & Toulmin, C. (2010). Food security: The challenge of feeding 9 billion people. Science, 327 (5967), 812–818.
Gurr, G. M., Lu, Z., Zheng, X., Xu, H., Zhu, P., Chen, G., Yao, X., Cheng, J., Zhu, Z., Catindig, J. L., & Villareal, S. (2016). Multi-country evidence that crop diversification promotes ecological intensification of agriculture. Nature Plants, 2 (3), 1–4.
Kaab, A., Sharifi, M., Mobli, H., Nabavi-Pelesaraei, A., & Chau, K. W. (2019). Combined life cycle assessment and artificial intelligence for prediction of output energy and environmental impacts of sugarcane production. Science of the Total Environment, 664 , 1005–1019.
Koop, S. H., & van Leeuwen, C. J. (2017). The challenges of water, waste and climate change in cities. Environment, Development and Sustainability, 19 (2), 385–418.
Koul, B., Yakoob, M., & Shah, M. P. (2022). Agricultural waste management strategies for environmental sustainability. Environmental Research, 206 , 112285.
Kumar, R., & Pal, P. (2015). Assessing the feasibility of N and P recovery by struvite precipitation from nutrient-rich wastewater: A review. Environmental Science and Pollution Research, 22 , 17453–17464.
Mariyono, J. (2020). Improvement of economic and sustainability performance of agribusiness management using ecological technologies in Indonesia. International Journal of Productivity and Performance Management, 69 (5), 989–1008.
Mohanty, A. K., Misra, M., & Drzal, L. T. (2002). Sustainable bio-composites from renewable resources: Opportunities and challenges in the green materials world. Journal of Polymers and the Environment, 10 , 19–26.
Moshontz, H., Campbell, L., Ebersole, C. R., IJzerman, H., Urry, H. L., Forscher, P. S., Grahe, J. E., McCarthy, R. J., Musser, E. D., Antfolk, J., & Castille, C. M. (2018). The Psychological Science Accelerator: Advancing psychology through a distributed collaborative network. Advances in Methods and Practices in Psychological Science, 1 (4), 501–515.
Moshou, D., Bravo, C., Oberti, R., West, J., Bodria, L., McCartney, A., & Ramon, H. (2005). Plant disease detection based on data fusion of hyper-spectral and multi-spectral fluorescence imaging using Kohonen maps. Real-Time Imaging, 11 (2), 75–83.
Neher, D. (2018). Ecological sustainability in agricultural systems: Definition and measurement. In Integrating sustainable agriculture, ecology, and environmental policy (pp. 51–61). Routledge.
Chapter Google Scholar
Nigussie, A., Kuyper, T. W., & de Neergaard, A. (2015). Agricultural waste utilisation strategies and demand for urban waste compost: Evidence from smallholder farmers in Ethiopia. Waste Management, 44 , 82–93.
Odebiri, O., Mutanga, O., Odindi, J., Peerbhay, K., & Dovey, S. (2020). Predicting soil organic carbon stocks under commercial forest plantations in KwaZulu-Natal province, South Africa using remotely sensed data. GIScience & Remote Sensing, 57 (4), 450–463.
Parawira, W. (2009). Biogas technology in sub-Saharan Africa: Status, prospects and constraints. Reviews in Environmental Science and Bio/Technology, 8 , 187–200.
Parfitt, J., Barthel, M., & Macnaughton, S. (2010). Food waste within food supply chains: Quantification and potential for change to 2050. Philosophical Transactions of the Royal Society B: Biological Sciences, 365 (1554), 3065–3081.
Pretty, J. (2008). Agricultural sustainability: Concepts, principles and evidence. Philosophical Transactions of the Royal Society B: Biological Sciences, 363 (1491), 447–465.
Reganold, J. P., & Wachter, J. M. (2016). Organic agriculture in the twenty-first century. Nature Plants, 2 (2), 1–8.
Sabiiti, E. N. (2011). Utilising agricultural waste to enhance food security and conserve the environment. African Journal of Food, Agriculture, Nutrition and Development, 11 (6), 1–9.
Sáez, J. A., Pérez-Murcia, M. D., Vico, A., Martínez-Gallardo, M. R., Andreu-Rodríguez, F. J., López, M. J., Bustamante, M. A., Sanchez-Hernandez, J. C., Moreno, J., & Moral, R. (2021). Olive mill wastewater-evaporation ponds long term stored: Integrated assessment of in situ bioremediation strategies based on composting and vermicomposting. Journal of Hazardous Materials, 402 , 123481.
Scarlat, N., Dallemand, J. F., & Fahl, F. (2018). Biogas: Developments and perspectives in Europe. Renewable Energy, 129 , 457–472.
Scarlat, N., Dallemand, J. F., Monforti-Ferrario, F., Banja, M., & Motola, V. (2015). Renewable energy policy framework and bioenergy contribution in the European Union–An overview from National Renewable Energy Action Plans and Progress Reports. Renewable and Sustainable Energy Reviews, 51 , 969–985.
Searchinger, T., Heimlich, R., Houghton, R. A., Dong, F., Elobeid, A., Fabiosa, J., Tokgoz, S., Hayes, D., & Yu, T. H. (2008). Use of US croplands for biofuels increases greenhouse gases through emissions from land-use change. Science, 319 (5867), 1238–1240.
Seidavi, A. R., Zaker-Esteghamati, H., & Scanes, C. G. (2019). Present and potential impacts of waste from poultry production on the environment. World’s Poultry Science Journal, 75 (1), 29–42.
Sheikh, M. R., Ali, N. A., & Aslam, A. (2022). Food wastage footprint, food security, environment and economic growth nexus in developing countries . InTech.
Shyamsundar, P., Springer, N. P., Tallis, H., Polasky, S., Jat, M. L., Sidhu, H. S., Krishnapriya, P. P., Skiba, N., Ginn, W., Ahuja, V., & Cummins, J. (2019). Fields on fire: Alternatives to crop residue burning in India. Science, 365 (6453), 536–538.
Siciliano, A., & Rosa, S. D. (2014). Recovery of ammonia in digestates of calf manure through a struvite precipitation process using unconventional reagents. Environmental Technology, 35 (7), 841–850.
Singh, Y., & Sidhu, H. S. (2014). Management of cereal crop residues for sustainable rice-wheat production system in the Indo-Gangetic plains of India. Proceedings of the Indian National Science Academy, 80 (1), 95–114.
Smith, P., Bustamante, M., Ahammad, H., Clark, H., Dong, H., Elsiddig, E. A., Haberl, H., Harper, R., House, J., Jafari, M., & Masera, O. (2014). Agriculture, forestry and other land use (AFOLU). In Climate change 2014: Mitigation of climate change (Contribution of Working Group III to the Fifth Assessment Report of the Intergovernmental Panel on Climate Change) (pp. 811–922). Cambridge University Press.
Sreekrishnan, T. R., Kohli, S., & Rana, V. (2004). Enhancement of biogas production from solid substrates using different techniques––A review. Bioresource Technology, 95 (1), 1–10.
Tripathi, N., Hills, C. D., Singh, R. S., & Atkinson, C. J. (2019). Biomass waste utilisation in low-carbon products: Harnessing a major potential resource. npj Climate and Atmospheric Science, 2 (1), 35.
Turner, W., Rondinini, C., Pettorelli, N., Mora, B., Leidner, A. K., Szantoi, Z., Buchanan, G., Dech, S., Dwyer, J., Herold, M., & Koh, L. P. (2015). Free and open-access satellite data are key to biodiversity conservation. Biological Conservation, 182 , 173–176.
Wahid, A., & Nararya, R. N. (2015). Optimasi Pengendalian Unit Gasifikasi dan Char Combustor pada Pabrik Biohidrogen dari Biomassa Menggunakan Reidentifikasi Model Predictive Control. In Prosiding Seminar Nasional Teknik Kimia Indonesia V (pp. 12–13). Universitas Gadjah Mada.
Wang, F., Zhao, H., Xiang, H., Wu, L., Men, X., Qi, C., Chen, G., Zhang, H., Wang, Y., & Xian, M. (2018). Species diversity and functional prediction of surface bacterial communities on aging flue-cured tobaccos. Current Microbiology, 75 , 1306–1315.
Westerman, P. W., & Bicudo, J. R. (2005). Management considerations for organic waste use in agriculture. Bioresource Technology, 96 (2), 215–221.
Wood, S. L., Jones, S. K., Johnson, J. A., Brauman, K. A., Chaplin-Kramer, R., Fremier, A., Girvetz, E., Gordon, L. J., Kappel, C. V., Mandle, L., & Mulligan, M. (2018). Distilling the role of ecosystem services in the Sustainable Development Goals. Ecosystem Services, 29 , 70–82.
Zhang, P., Li, Z., Ghardallou, W., Xin, Y., & Cao, J. (2023). Nexus of institutional quality and technological innovation on renewable energy development: Moderating role of green finance. Renewable Energy, 214 , 233–241.
Zhang, X., He, L., Zhang, J., Whiting, M. D., Karkee, M., & Zhang, Q. (2020). Determination of key canopy parameters for mass mechanical apple harvesting using supervised machine learning and principal component analysis (PCA). Biosystems Engineering, 193 , 247–263.
Download references
Author information
Authors and affiliations.
Department of Applied Science & Humanities, Darbhanga College of Engineering, Darbhanga, Bihar, India
Dipti Bharti & Rahul Singh
Department of Chemistry, Noida International University, Greater Noida, Uttar Pradesh, India
Abhilekha Sharma
Department of Chemistry, IEC University, Baddi, Himachal Pradesh, India
Meenakshi Sharma
Department of Mechanical Engineering, Indian Institute of Technology, Ropar, Punjab, India
Department of Biotechnology, Invertis University, Bareilly, Uttar Pradesh, India
Richa Saxena
You can also search for this author in PubMed Google Scholar
Editor information
Editors and affiliations.
Chitkara University School of Engineering and Technology, Chitkara University, Solan, Himachal Pradesh, India
Arun Lal Srivastav
School of Life Sciences, Amity University Madhya Pradesh, Gwalior, Madhya Pradesh, India
Abhishek Kumar Bhardwaj
Sardar Vallabhbhai Patel University of Agriculture and Technology, Meerut, Uttar Pradesh, India
Mukesh Kumar
Rights and permissions
Reprints and permissions
Copyright information
© 2024 The Author(s), under exclusive license to Springer Nature Switzerland AG
About this chapter
Bharti, D., Sharma, A., Sharma, M., Singh, R., Kumar, A., Saxena, R. (2024). Cultivating a Greener Tomorrow: Sustainable Agriculture Strategies for Minimizing Agricultural Waste. In: Srivastav, A.L., Bhardwaj, A.K., Kumar, M. (eds) Valorization of Biomass Wastes for Environmental Sustainability. Springer, Cham. https://doi.org/10.1007/978-3-031-52485-1_18
Download citation
DOI : https://doi.org/10.1007/978-3-031-52485-1_18
Published : 15 March 2024
Publisher Name : Springer, Cham
Print ISBN : 978-3-031-52484-4
Online ISBN : 978-3-031-52485-1
eBook Packages : Earth and Environmental Science Earth and Environmental Science (R0)
Share this chapter
Anyone you share the following link with will be able to read this content:
Sorry, a shareable link is not currently available for this article.
Provided by the Springer Nature SharedIt content-sharing initiative
- Publish with us
Policies and ethics
- Find a journal
- Track your research
- Open access
- Published: 15 March 2022
Effects of sustainable agricultural practices on farm income and food security in northern Ghana
- Edinam Dope Setsoafia ORCID: orcid.org/0000-0001-7213-8920 1 ,
- Wanglin Ma 1 &
- Alan Renwick ORCID: orcid.org/0000-0001-7847-8459 1
Agricultural and Food Economics volume 10 , Article number: 9 ( 2022 ) Cite this article
13k Accesses
37 Citations
Metrics details
The adoption of sustainable agricultural practices (SAPs) has been recommended by many experts and international institutions to address food security and climate change problems. Global support for the Sustainable Development Goals has focused attention on efforts to up-scale the adoption of SAPs in developing countries where growth in populations and incomes compromises the resilience of natural resources. This study investigates the factors affecting smallholder farmers’ decisions to adopt SAPs (improved seed, fertilizer, and soil and water conservation) and the impacts of the adoption on farm income and food security, using data collected from Ghana. Food security is captured by the reduced coping strategy index and household dietary diversity. The multinomial endogenous switching regression model is utilized to address selection bias issues. Results show that farmers’ decisions to adopt SAPs are influenced by the social demographics of the households, plot-level characteristics, extension services and locations. Adopting all three SAPs has larger positive impacts on farm income and food security than adopting single or two SAPs. Our findings advocate for policies that enhance the quality of extension service and strengthen farmer-based organizations for the wider dissemination of adequate SAP information. Farmers should be encouraged to adopt SAPs as a comprehensive package for increasing farm income and ensuring food security.
Introduction
There is considerable pressure on agriculture to meet the demands of a growing world population. This is heightened with rising demand for necessities such as food, raw materials for industries, and biofuels. However, growth in agricultural production globally does not match this demand well, especially in parts of Africa. Africa has been projected to be vulnerable to climate change because of its proximity to the equator (Ojo et al. 2021 ; Thinda et al. 2021 ; Sarr et al. 2021 ; Onyeneke 2021 ; Ahmed 2022 ). Some of the physical impacts of climate change in Africa are rising sea levels, temperature andchange, and rainfall change (World Bank 2010 ; Abdulai 2018 ), which will harm agricultural productivity, farm income, food security, and economic development. This will negatively affect the poor, whose livelihoods are tired of agriculture in Sub-Saharan Africa.
There has been a global discussion on overcoming the negative externalities of climate change. Most experts believe that sustainable agriculture management could be a solution to the challenge associated with climate change (Kassie et al. 2013 ; Ndiritu et al. 2014 ; Ogemah 2017 ; Zhou et al. 2018 ; Adenle et al. 2019 ; Rose et al. 2019 ; Zeweld et al. 2020 ; Ma and Wang 2020 ; Ehiakpor et al. 2021 ; Bekele et al. 2021 ). This approach is expected to improve agricultural production performance whilst reversing the negative degradation processes on the agroecosystem, particularly in smallholder farming systems. It is an upgrade of the green revolution, which led to a significant increase in agricultural productivity globally and is credited for jump-starting economies in Asia out of poverty but has left negative externalities such as deforestation, land degradation, salinization of water bodies, and loss of biodiversity in its wake.
To reverse the negative externalities from crop intensification, farmers have been advised to adopt sustainable agricultural practices (SAPs), which are made up of elements of the green revolution and an agronomic revolution. The literature is filled with studies on the adoption of specific or single elements of SAPs, such as improved seed, irrigation, drought-tolerant crop varieties, climate-resilient crop variety, organic soil amendments, and soil and water conservation practices, and their effects on crop yield and net farm income (Abdulai and Huffman 2014 ; Agula et al. 2018 ; Adenle et al. 2019 ; Adegbeye et al. 2020 ; Kimathi et al. 2021 ; Zheng et al. 2021 ; Ahmed 2022 ; Yang et al. 2022 ). Despite the potential complementarity or substitutability of specific elements of SAPs, the research on the adoption of multiple SAPs and their effects on outcome variables such as income, outputs, consumption expenditure and food security remains limited.
This paper seeks to investigate the determinants of multiple SAP adoption and the adoption effects on farm income and food security, using second-hand data collected from Ghana. This study contributes to the literature in twofold. First, it provides empirical insights into the importance of SAPs on welfare indicators, specifically food security. The use of food security as a proxy measure for welfare is particularly important in the Ghanaian context, where farming is done mostly on a subsistence level, and farmers sell crops as and when they need cash. Thus, farmers may be food secure but not have a high net farm income or high consumption expenditure. Our analysis extends previous studies that have focused on other proxies of household welfare such as net farm income, net crop income and consumption expenditure (Kassie et al. 2013 ; Teklewold et al. 2013a ; Manda et al. 2016 ; Bopp et al. 2019 ; Oyetunde Usman et al. 2020 ; Ehiakpor et al. 2021 ). Secondly, we employ a multinomial endogenous switching regression model to mitigate selection bias. In particular, this model helps address the selection bias issues arising from observed factors (e.g., age, gender and education) and unobserved factors (farmers’ innate ability in innovation adoption and motivations to address external shocks). Findings from the study will aid in formulating specific policies targeted at improving SAP adoption and enhancing the food security status of farm households in developing countries.
The remaining sections of the paper are as follows; " Literature review " section covers a review of relevant literature. The methodology is presented in " Methodology " section. The descriptive and empirical results are presented and discussed in " Results and discussions " section. The final section highlights the conclusions and policy implications of the findings.
Literature review
A growing number of studies have explored the factors that determine the adoption of SAPs in Africa. In the past, most of the works have focused on single components of SAPs (Abdulai and Huffman 2014 ; Carrión Yaguana et al. 2015 ; Fisher et al. 2015 ; Adenle et al. 2019 ; Manda et al. 2020a ; Martey et al. 2020 ; Kimathi et al. 2021 ; Lampteym 2022 ). For example, Abdulai and Huffman ( 2014 ) reported that rice farmers’ decisions to adopt soil and water conservation are influenced by their education, capital and labour constraints, social networks, extension contacts, and farm soil conditions. Manda et al. ( 2018 ) found that farmers’ decisions to adopt improved maize varieties are mainly influenced by education, household size, livestock holdings, land per capita, market information, and locations in Zambia. The study by Martey et al. ( 2020 ) reveals that farmers’ adoption of drought-tolerant maize varieties is mainly determined by access to seed, gender, access to extension, labour availability and location of the farmer in Ghana. Kimathi et al. ( 2021 ) investigated farmers’ decisions to adopt climate-resilient potato varieties and found that the main factors affecting adoption were access to information, quality seeds, training, group membership and variations in agro-ecological zones.
Some studies have also explored the factors affecting smallholder farmers’ decisions to adopt multiple SAPs. Most of the past works have been focused on Eastern and Southern Africa (Teklewold et al. 2013a ; Kassie et al. 2015 ; Cecchini et al. 2016 ; Bese et al. 2021 ; Nonvide 2021 ), though a growing number of studies seek to bridge the research gap in the adoption of multiple SAPs in West Africa (Nkegbe and Shankar 2014 ; Struik et al. 2014 ; Ehiakpor et al. 2021 ; Faye et al. 2021 ). The multiple SAPs considered by Teklewold et al. ( 2013a ) include maize–legume rotation, conservation tillage, animal manure use, improved seed, and inorganic fertiliser use. They showed that a household’s trust in government support, credit constraints, spouse education, rainfall and plot-level disturbances, household wealth, social capital and networks, labour availability, plot and market access are the main factors determining both the probability and the extent of adoption of SAPs in rural Ethiopia. In their investigation for Ghana, the multiple SAPs considered by Ehiakpor et al. ( 2021 ) include improved maize seeds, maize-legume rotation, animal manure, legume intercropping, crop residue retention, zero/minimum tillage, integrated pest management, and chemical fertilizer. Non-farm income, livestock ownership, pest and disease prevalence, farmers’ experience of erosion, farmers’ perception of poor soil fertility, participation in field demonstration, membership of saving groups, access to agricultural credit, plot ownership, and distance to the agricultural input market are found to be important determinants of adoption of SAPs (Ehiakpor et al. 2021 ).
Studies estimating the impacts of SAP have utilized various outcome variables, such as household income, agrochemical use, demand for labour, crop yields, food security (Teklewold et al. 2013b ; Abdulai and Huffman 2014 ; Gebremariam and Wünscher 2016 ; Manda et al. 2016 ; Amondo et al. 2019 ; Marenya et al. 2020 ; Oduniyi and Chagwiza 2021 ). Gebremariam and Wünscher ( 2016 ) found that higher combinations of SAPs led to higher payoff measured by net crop income and consumption expenditure in Ghana. Khonje et al. ( 2018 ) showed that joint adoption of multiple SAPs had higher impacts on yields, household income and poverty than the adoption of components of the technology package in Zambia. Amondo et al. ( 2019 ) found that adopting drought-tolerant maize varieties increases maize yield by 15% in Zambia. Marenya et al. ( 2020 ) concluded that a higher number of SAPs adopted resulted in higher maize grain yield and maize income in Ethiopia. The adoption of elements of SAPs has been said to be context-specific because there are no blueprints of the various combination of SAPs that work in every environment. Therefore, this study explores how SAP adoption affects farm income and food security, using Ghana as a case.
Methodology
Smallholder farmers make decisions to adopt SAPs in response to external shocks such as drought, erosion, perceived decline in soil fertility, weeds, pests, and diseases. Both observed factors (e.g., age, gender, education and farm size) and unobserved factors (e.g., farmers’ innate abilities and motivations) may affect their decisions when choosing to adopt a single SAP or a package (Kassie et al. 2013 ; Teklewold et al. 2013a ; Manda et al. 2016 ; Ehiakpor et al. 2021 ). Due to the self-selection nature of technology adoption, farmers without adopting any SAPs and those adopting a single SAP or package may be systematically different. The fact results in a selection bias issue, which should be addressed for consistently estimating the effects of SAP adoption.
When technology adoption has more than two options, previous studies have used either the multi-valued treatment effects (MVT) model (Cattaneo 2010 ; Ma et al. 2021 ; Czyżewski et al. 2022 ) or the multinomial endogenous switching regression (MESR) model (Kassie et al. 2015 ; Oparinde 2021 ; Ahmed 2022 ) to address the selection bias issues. For example,Czyżewski et al. ( 2022 ) estimated the long-term impacts of political orientation (economic views and individual value systems) on the environment using the MVT model. They confirmed that local orientation is conducive to long-term environmental care. Using the MESR model, Ahmed ( 2022 ) evaluated the impact of improved maize varieties and inorganic fertilizer on productivity and wellbeing. He found that combining the two technologies significantly boosts maize yield and consumption expenditure than adopting the technologies in isolation. Because of the non-parametric nature, the MVT model can only address the observed selection bias and does not account for unobserved section bias. In comparison, the MESR model can help mitigate selection bias issues arising from both observed and unobserved factors, and thus, it is employed in this study.
Multinomial endogenous switching regression
The MESR model estimate three stages. The first stage models factors affecting smallholder farmers’ decisions to adopt a specific SAP technology or a package. Following Teklewold et al. ( 2013a ), this study focuses on three main SAP technologies, namely improved seeds (I), fertilizer (F), and soil and water conservation (cereal-legume rotation/cereal – legume intercropping, manure use, organic input use) (S). The three categories result in eight possible choices of SAPs. It bears an emphasis here that because of the small number of observations in the group that captures the combination of improved seed and fertilizer (26 samples) and the group that captures the combination of improved seed and soil and water conservation (9 samples), we combined them in empirical estimations. Also, it is worth noting here that no household has only adopted improved seed. These facts indicate that there are six mutually exclusive choices of SAP technology, including (1) non-adoption (I 0 F 0 S 0 ); (2) fertilizer only (I 0 F 1 S 0 ); (3) soil and water conservation only (I 0 F 0 S 1 ); (4) combination of improved seed and fertilizer and combination of improved seed and soil and water conservation (I 1 F 1 S 0 ); (5) combination of fertilizer and soil and water conservation (I 0 F 1 S 1 ); (6) combination of improved seed, fertilizer, and soil and water conservation (I 1 F 1 S 1 ). Farmers choose one of the six possible choices to maximize the expected benefit.
The study assumes that the error terms are identical and independently Gumbel distributed, the probability that farmer i , with X characteristics will choose package j, is specified using a multinomial logit model (McFadden 1973 ; Teklewold et al. 2013a ; Zhou et al. 2020 ; Ma et al. 2022b ). It is specified as follows:
where P ij represents the probability that a farmer i chooses to adopt SAP technology j. X i is a vector of observed exogenous variables that capture household, plot, and location-level characteristics. β j is a vector of parameters to be estimated. The maximum likelihood estimation is used to estimate the parameters of the latent variable model.
In the second stage, the ordinary least square (OLS) model is used to establish the relationship between the outcome variables (farm income and food security) and a set of exogenous variables denoted by Z for the chosen SAP technology. Non-adoption of SAPs (i.e., base category, I 0 F 0 S 0 ) is denoted as j = 1, with the other combinations denoted as ( j = 2 …, 6). The possible equations for each regime is specified as:
where I is an index that denotes farmer i ’s choice of adopting a type of SAP technology; Q i is the outcome variables for the i- th farmer; Z i is a vector of exogenous variables; α 1 and α J are parameters to be estimated; u i 1 and u iJ are the error terms.
Relying on a vector of observed covariates, captured by Z i , Eqs. (2a) and (2b) can help address the observed selection bias issue. However, if the same unobserved factors (e.g., farmers’ motivations to adopt SAPs) simultaneously influence farmers’ decisions to adopt SAPs and outcome variables, the error terms in Eqs. (2a) and (2b) and the error term in Eq. ( 1 ) would be correlated. In this case, unobserved selection bias occurs. Failing to address such type of selection bias would generate biased estimates. Within the MESR framework, the selectivity correction terms are calculated after estimating Eq. ( 1 ) and then included into Eqs. (2a) and (2b) to mitigate unobserved selection bias. Formally, Eqs. (2a) and (2b) can be rewritten as follows:
where Q i and Z i are defined earlier; λ 1 and λ J are selectivity correction terms used to address unobserved selection bias issues; σ 1 and σ J are covariance between error terms in Eqs. ( 1 ), (2a) and (2b). In the multinomial choice setting, there are J − 1 selectivity-correction terms, one for each alternative SAP combination.
For consistently estimating the MESR model, at least one instrumental variable (IV) should be included in X i in the MNL model but not in the Z i in the outcome equations. In this study, two distance variables, distance to weekly market and minutes 30 to the plot, are employed as IVs for model identification purposes. Distance to the weekly market is measured as a continuous variable, measured in minutes. The variable representing minutes 30 to plot is a dummy variable, which equals 1 if the plot is within 30 min from the homestead and 0 otherwise. The two IVs are not expected to affect farm income and food security directly. We checked the validity of the IVs by running the Falsification test and conducting the correlation coefficient analysis (Pizer 2016 ; Liu et al. 2021 ; Ma et al. 2022a ). For the sake of simplicity, we did not report the results.
The average treatment effect on the treated (ATT) is calculated at the third step. This involves comparing the expected outcomes (farm income and food security) of SAP adopters and non-adopters, with and without adoption. Using experimental data, it is easier to establish impacts; however, this study is based on observational cross-sectional data, thus making impact evaluation a bit challenging. The challenge is mainly estimating the counterfactual outcome, i.e. the outcome of SAP adopters if they had not adopted the SAP technology. Following previous studies (Kassie et al. 2015 ; Oparinde 2021 ; Ahmed 2022 ), the study estimates ATT in the actual and the counterfactual scenarios using the following equations:
The outcome variables for SAP adopters with adoption (observed):
The outcome variables for SAP adopters had they decided not to adopt (Counterfactual):
The difference between Eqs. (4a) and (5a) or Eqs. (4b) and (5b) is the ATT. For example, the difference between Eqs. (4a) and (5a) is given as:
Data and variables
The study used data collected by IITA for their Africa RISING project ( https://africa-rising.net/ ) in the three northern regions, namely, Northern, Upper East, and Upper West regions. The data was collected in 2014 from 1284 households operating approximately 5500 plots in 50 rural communities in northern Ghana. The baseline survey used a stratified two-stage sampling technique, and data was collected using Computer Assisted Personal Interviewing (CAPI) supported by Survey CTO software on tablets (Tinonin et al. 2016 ). A structured questionnaire was used to conduct the household interviews. The data covers the various SAP technologies, demographic characteristics, agricultural land holdings, crop outputs and sales, livestock production, farmers’ access to agricultural information and knowledge, access to credit and markets, household assets, and income.
The outcome variables for this study are farm income and food security. The farm income of crops cultivated is obtained by valuing the yield of crops at market price and deducting the costs of all variable inputs. Two variables capture food security, including reduced coping strategy index (rCSI) and household dietary diversity (HDD). Specifically, the rCSI is an index that is measured by scoring coping strategies households use (and frequency of use) when they experience food insecurity. rCSI is an index with five standardized questions on the coping strategies used when faced with food insecurity, the more strategies used, and food insecure the household is. The rCSI score ranges from 0 to 63. A higher level of rCSI score means a higher level of food insecurity. The HDD variable is based on the diverse food groups a household consumes. The higher the score, the more diverse the diet of a household, and the more food secure the household is. Drawing upon previous empirical studies on the adoption of SAPs and related agricultural innovations (Kassie et al. 2013 ; Teklewold et al. 2013a ; Manda et al. 2016 ; Bopp et al. 2019 ; Oyetunde Usman et al. 2020 ; Ma and Wang 2020 ; Ehiakpor et al. 2021 ; Pham et al. 2021 ), we have identified and selected a range of control variables that may influence the adoption of SAPs. These include age, gender, education, marital status, household size, farm size, off-farm income, Africa RISING member, extension, extension satisfaction, number of crops, drought and floods, market access, sandy soil, clay soil, flat slope, moderate to steep, and location dummies.
Results and discussions
Descriptive results.
Table 1 shows the frequency of respondents that used the different categories of SAPs. Of the eight possible categories of SAPs initially specified, 6.78% of farmers in our sample did not adopt any SAPs (I 0 F 0 S 0 ). No farmers adopted imported seed only (I 1 F 0 S 0 ), while only 9 farmers combined improved seed and soil and water conversation as SAPs (I 1 F 0 S 1 ). Only 26 farmers combined improved seed and soil and water conservation as SAPs (I 1 F 1 S 0 ). Therefore, as discussed earlier, we merged I 1 F 1 S 0 and I 1 F 0 S 1 into one group (coded as I 1 F 1 S 0 ), and the empirical analysis includes six groups in total. Table 1 also shows that more than half of the farmers in our sample (51.17%) combined fertilizer and soil and water conservation as SAPs. Around 7% of farmers adopted all the three identified SAPs.
Table 2 presents the variables and statistical descriptions. It shows that the average farm income is 2561 GHS (roughly 400 USD). The average means of rCSI and HDD, which capture food security, are 5.576 and 7.799, respectively. Table 2 also shows that the average age of respondents was about 48 years. Around 84% of respondents are male, and almost 90% of respondents got married. The surveyed households averagely have around 9 persons. About 61% of respondents received advice from extension officers, and 45.6% were satisfied with the extension services. Approximately 70% of respondents had accessed the markets.
Empirical results
Determinants of adoption of sap categories.
Table 3 presents the results estimated by the MNL model, demonstrating the factors that influence smallholder farmers’ decisions to adopt different SAPs categories. Farmers without adopting any type of SAPs (i.e. I 0 F 0 S 0 ) are used as the reference group in empirical estimations. Because the primary objective of the MNL model estimations is to calculate the selectivity correction terms rather than explain the determinants of SAP adoption perfectly, we explain the results of Table 3 briefly. The results show gender variable has significant coefficients in columns 2, 4 and 5. Our results appear to suggest that women are more likely to combine improved seeds and fertilizer (I 1 F 1 S 0 ) as SAPs to increase farm productivity. In comparison, men are more likely to rely on fertilizer (I 0 F 1 S 0 ) or combine fertilizer and soil and water conservation technology ( I 0 F 1 S 1 ) as SAPs to improve farm performance. Our findings are largely supported by the previous studies (Smale et al. 2018 ; Paudel et al. 2020 ; Tambo et al. 2021 ), reporting gendered differences in agricultural technology adoption. For example, Smale et al. ( 2018 ) found that women are more likely to adopt improved seeds on the plots they manage in Sudan. Education has positive impacts in all estimated specifications but is only statistically significant in the specification of adopting improved seed and fertilizer (I 1 F 1 S 0 ). Better education enables farmers to be aware of the benefits of SAPs and motivate them to adopt them, especially productivity-enhancing technologies such as improved seed and fertilizer. This finding is consistent with the findings of Kassie et al. ( 2014 ) for Tanzania and Gebremariam and Wünscher ( 2016 ) for Ghana.
The significant coefficients of household size in columns 2 and 6 suggest that larger households are more likely to adopt multiple SAPs (I 1 F 1 S 1 ) but are less likely to adopt single SAP such as fertilizer (I 0 F 1 S 0 ). Larger households usually mean better labour endowments, allowing them to adopt multiple SAPs more easily than small ones. This is consistent with the findings of Kassie et al. ( 2014 ). Off-farm income has positive and significant coefficients in columns 3, 5 and 6. The findings suggest that farmers receiving a higher level of off-farm income are more likely to adopt fertilizer only (I 0 F 1 S 0 ), combine fertilizer and soil and water conservation as SAPs (I 0 F 1 S 1 ), and adopt all three SAPs (I 1 F 1 S 1 ). Additional income from off-farm activities can help release credit constraint issues, allowing farmers to invest in innovative technologies such as SAPs to improve farm performance. In their study for Pakistan, Kousar and Abdulai ( 2016 ) found that participation in off-farm work increases farmers’ adoption of soil conservation measures.
The African RISING member variable has a positive and statistically significant impact on farmers’ fertiliser adoption only (I 0 F 1 S 0 ), the combination of improved seed and fertilizer (I 1 F 1 S 0 ), and the combination of fertilizer and soil and water conservation (I 0 F 1 S 1 ). The importance of farmer-based organisations in promoting the adoption of innovative technologies has been widely discussed in the literature (Zhang et al. 2020 ; Manda et al. 2020b ; Yu et al. 2021 ). For example, Manda et al. ( 2020a , b ) reported that membership in agricultural cooperatives increases the adoption speed of improved maize by 1.6–4.3 years. We show that farmers having access to extension services are more likely to adopt SAPs, including fertilizer only (I 0 F 1 S 0 ), soil and water conservation only (I 0 F 0 S 1 ), and all three SAps (I 1 F 1 S 1 ). In their studies for Nepal, Suvedi et al. ( 2017 ) found that farmers’ participation in extension programs increases their adoption of improved crop varieties. This finding is further confirmed by Nakano et al. ( 2018 ), who found that farmer-to-farmer training through extension programs enhance farmers’ adoption of technologies (e.g., fertilizer and improved bund) in Tanzania. The location dummies are statistically significant in columns 2, 4 and 5. Our findings suggest that relative to farmers living in Upper West (reference group), those residing in Northern and Upper East are more likely to adopt fertilizer only (I 0 F 1 S 0 ) and a combination of fertilizer and soil and water conservation (I 0 F 1 S 1 ), but less likely to adopt the combination of improved seeds and fertilizer (I 1 F 1 S 0 ). Our findings confirm spatial-fixed characteristics (e.g., social-economic conditions, resource endowments, climate conditions, and institutional arrangements) may also affect smallholder farmers’ decisions to adopt SAPs and highlight the importance of including them in estimations.
Average treatment effects of SAPs
Table 4 presents the results estimating the treatment effects of SAP adoption on farm income and food security. For the sake of brevity, we do not present and discuss the results estimated by the OLS regression model but are available upon reasonable requests. Our ATT estimate results in Table 4 record differentiated findings regarding the impacts of adopting only one SAP technology on farm income and food security, measured by rCSI score and HDD score. Specifically, adopting only fertilizer (I 0 F 1 S 0 ) significantly reduces rCSI score and improves HDD score. The ATT estimates indicate that fertilizer adoption only (I 0 F 1 S 0 ) decreases rCSI score by 42% and increases the HDD score by 6.5%. We find that fertilizer adoption only (I 0 F 1 S 0 ) decreases farm income. A possible reason could be the improper use of fertilizer by smallholder farmers, such as using lower than recommended amounts of fertilizer; hence they do not achieve the maximum potential output expected.
Adoption of SAP package that combines improved seed and fertilizer (I 1 F 1 S 0 ) improves food security significantly. The ATT estimates show that I 1 F 1 S 0 adoption reduces rCSI score by 45% and increases HDD score by 4%. However, I 1 F 1 S 0 adoption decreases farm income, a finding that is largely consistent with the finding of Ma and Wang ( 2020 ), showing that SAP adoption significantly decreases farm income in China. Adoption of SAP package that combines fertilizer and soil and water conservation (I 0 F 1 S 1 ) increases farm income and improves food security. We show that I 0 F 1 S 1 adoption increases farm income by 12%, reduces rCSI score by 23%, and improves HDD score by 5%.
The ATT estimates show that adopting all the three SAPs (I 1 F 1 S 1 ) positively and statistically impacts farm income and food security. The impact magnitudes of adopting all the three SAPs are larger than that of adopting single or two SAPs. Specifically, the I 1 F 1 S 1 adoption increases farm income by 23%, reduces rCSI score by 53%, and improves HDD score by 14%. Our results are largely supported by the previous studies (Teklewold et al. 2013a ; Manda et al. 2016 ; Oduniyi and Chagwiza 2021 ), pointing out that adopting multiple SAPs has larger impacts on welfare measures than adopting only one or two SAPs. For example, Teklewold et al. ( 2013b ) showed that multiple SAP adoption significantly increases household income in Ethiopia. Oduniyi and Chagwiza ( 2021 ) found that adopting sustainable land management practices increases the food security of smallholder farmers in South Africa.
Conclusions and policy implications
Many institutions have credited sustainable agricultural practices (SAPs) as a viable solution that helps tackle the worlds’ feeding problems and worsening environmental issues. This study used a multinomial endogenous switching regression (MESR) to investigate factors that affect smallholder farmers’ decisions to adopt different categories of SAPs and estimate the effects of the adoption on farm income and food security. In particular, we used two measures, including rCSI score and HDD score, to capture food security. We estimated the data collected by IITA for their Africa RISING project in Ghana.
The MNL results showed that farmers’ decisions to adopt SAPs are influenced by the social demographics of the households (e.g., gender, education, marital status, and household size), plot-level characteristics (e.g., number of crops, soil types, and topography), extension services, and locations. The study also recorded differentiated findings regarding the impacts of adopting only one or two SAPs on farm income and food security. For example, adopting only fertilizer significantly reduces rCSI score and improves HDD score, but it unexpectedly decreases farm income. Adoption of SAP package that combines improved seed and fertilizer significantly improves food security measures, but it also decreases farm income. Nevertheless, we found that adopting all the three SAPs positively and statistically impacts farm income and food security. The impact magnitudes of adopting all the three SAPs are larger than that of adopting single or two SAPs.
The study highlights that policies that improve the extension agents to farmer ratio should be pursued since access to extension positively influenced the adoption of SAPs. The satisfaction with the extension agent variable positively influenced the adoption of all the SAPs. This highlights the need to improve the quality of extension service to minimize the risk of adoption due to inadequate information transfer. Membership in farmer-based organizations (FBOs) such as Africa RISING positively influenced the adoption of different packages of SAPs. Therefore farmers should be encouraged to join FBOs, and similar organizations should be established or strengthened to enhance the dissemination of information regarding SAPs. Policies to improve farmer income and food security should advocate for the comprehensive adoption of all the SAPs packages and provide incentives to motivate the adoption of all SAPs packages.
Availability of data and materials
Data is available from the leading author upon the reasonable request.
Abdulai A (2018) Simon Brand Memorial Address: The challenges and adaptation to climate change by farmers in sub-Saharan Africa. Agrekon 57:28–39. https://doi.org/10.1080/03031853.2018.1440246
Article Google Scholar
Abdulai A, Huffman W (2014) The adoption and impact of soil and water conservation technology: an endogenous switching regression application. Land Econ 90:26–43
Adegbeye MJ, Reddy PRK, Obaisi AI et al (2020) Sustainable agriculture options for production, greenhouse gasses and pollution alleviation, and nutrient recycling in emerging and transitional nations—an overview. J Clean Prod 242:118–319
Adenle AA, Wedig K, Azadi H (2019) Sustainable agriculture and food security in Africa: the role of innovative technologies and international organizations. Technol Soc 58:101143
Agula C, Akudugu MA, Dittoh S, Mabe FN (2018) Promoting sustainable agriculture in Africa through ecosystem-based farm management practices: evidence from Ghana. Agric Food Secur 7:5
Ahmed MH (2022) Impact of improved seed and inorganic fertilizer on maize yield and welfare: evidence from Eastern Ethiopia. J Agric Food Res 7:100266. https://doi.org/10.1016/j.jafr.2021.100266
Amondo E, Simtowe F, Rahut DB, Erenstein O (2019) Productivity and production risk effects of adopting drought-tolerant maize varieties in Zambia. Int J Clim Chang Strateg Manag 11:570–591. https://doi.org/10.1108/IJCCSM-03-2018-0024
Bekele RD, Mirzabaev A, Mekonnen D (2021) Adoption of multiple sustainable land management practices among irrigator rural farm households of Ethiopia. L Degrad Dev 32:5052–5068. https://doi.org/10.1002/ldr.4091
Bese D, Zwane E, Cheteni P (2021) The use of sustainable agricultural methods amongst smallholder farmers in the Eastern Cape province, South Africa. Afr J Sci Technol Innov Dev 13:261–271. https://doi.org/10.1080/20421338.2020.1724388
Bopp C, Engler A, Poortvliet PM, Jara-Rojas R (2019) The role of farmers’ intrinsic motivation in the effectiveness of policy incentives to promote sustainable agricultural practices. J Environ Manage 244:320–327. https://doi.org/10.1016/j.jenvman.2019.04.107
Carrión Yaguana V, Alwang J, Norton G, Barrera V (2015) Does IPM have staying power? Revisiting a potato-producing area years after formal training ended. J Agric Econ 66:1–16
Cattaneo MD (2010) Efficient semiparametric estimation of multi-valued treatment effects under ignorability. J Econom 155:138–154. https://doi.org/10.1016/j.jeconom.2009.09.023
Cecchini S, Scott C, Imai KS et al (2016) Does adaptation to climate change provide food security? A micro-perspective from Ethiopia. Am J Agric Econ 46:825–842. https://doi.org/10.1093/ajae/aar006
Czyżewski B, Polcyn J, Brelik A (2022) Political orientations, economic policies, and environmental quality: multi-valued treatment effects analysis with spatial spillovers in country districts of Poland. Environ Sci Policy 128:1–13. https://doi.org/10.1016/j.envsci.2021.11.001
Ehiakpor DS, Danso-Abbeam G, Mubashiru Y (2021) Adoption of interrelated sustainable agricultural practices among smallholder farmers in Ghana. Land use policy 101:105142
Faye JB, Hopple AM, Bridgham SD (2021) Indigenous farming practices increase millet yields in Senegal, West Africa. Agroecol Sustain Food Syst 45:159–174. https://doi.org/10.1080/21683565.2020.1815927
Fisher M, Abate T, Lunduka RW et al (2015) Drought tolerant maize for farmer adaptation to drought in sub-Saharan Africa: determinants of adoption in eastern and southern Africa. Clim Change 133:283–299. https://doi.org/10.1007/s10584-015-1459-2
Gebremariam G, Wünscher T (2016) Combining sustainable agricultural practices pays off: evidence on welfare effects from Northern Ghana. African Association of Agricultural Economists (AAAE)
Kassie M, Jaleta M, Shiferaw B et al (2013) Adoption of interrelated sustainable agricultural practices in smallholder systems: evidence from rural Tanzania. Technol Forecast Soc Change 80:525–540. https://doi.org/10.1016/j.techfore.2012.08.007
Kassie M, Jaleta M, Mattei A (2014) Evaluating the impact of improved maize varieties on food security in Rural Tanzania: evidence from a continuous treatment approach. Food Secur 6:217–230. https://doi.org/10.1007/s12571-014-0332-x
Kassie M, Teklewold H, Jaleta M et al (2015) Understanding the adoption of a portfolio of sustainable intensification practices in eastern and southern Africa. Land Use Policy 42:400–411. https://doi.org/10.1016/j.landusepol.2014.08.016
Khonje MG, Manda J, Mkandawire P et al (2018) Adoption and welfare impacts of multiple agricultural technologies: evidence from eastern Zambia. Agric Econ 49:599–609. https://doi.org/10.1111/agec.12445
Kimathi SM, Ayuya OI, Mutai B (2021) Adoption of climate-resilient potato varieties under partial population exposure and its determinants: Case of smallholder farmers in Meru County, Kenya. Cogent Food Agric 7:66. https://doi.org/10.1080/23311932.2020.1860185
Kousar R, Abdulai A (2016) Off-farm work, land tenancy contracts and investment in soil conservation measures in rural Pakistan. Aust J Agric Resour Econ 60:307–325
Lampteym S (2022) Agronomic practices in soil water management for sustainable crop production under rain fed agriculture of drylands in sub-Sahara Africa. Afr J Agric Res 18:18–26. https://doi.org/10.5897/AJAR2021.15822
Liu M, Min S, Ma W, Liu T (2021) The adoption and impact of E-commerce in rural China: application of an endogenous switching regression model. J Rural Stud 83:106–116. https://doi.org/10.1016/j.jrurstud.2021.02.021
Ma W, Wang X (2020) Internet use, sustainable agricultural practices and rural incomes: evidence from China. Aust J Agric Resour Econ 64:1087–1112. https://doi.org/10.1111/1467-8489.12390
Ma W, Zhu Z, Zhou X (2021) Agricultural mechanization and cropland abandonment in rural China. Appl Econ Lett 00:1–8. https://doi.org/10.1080/13504851.2021.1875113
Ma W, Vatsa P, Zhou X, Zheng H (2022a) Happiness and farm productivity: insights from maize farmers in China. Int J Soc Econ 49:97–106. https://doi.org/10.1108/IJSE-08-2021-0474
Ma W, Zheng H, Gong B (2022b) Rural income growth, ethnic differences, and household cooking fuel choice: evidence from China. Energy Econ 107:105851. https://doi.org/10.1016/j.eneco.2022.105851
Manda J, Alene AD, Gardebroek C et al (2016) Adoption and impacts of sustainable agricultural practices on maize yields and incomes: evidence from rural Zambia. J Agric Econ 67:130–153. https://doi.org/10.1111/1477-9552.12127
Manda J, Gardebroek C, Kuntashula E, Alene AD (2018) Impact of improved maize varieties on food security in Eastern Zambia: a doubly robust analysis. Rev Dev Econ 22:1709–1728. https://doi.org/10.1111/rode.12516
Manda J, Alene AD, Tufa AH et al (2020a) Adoption and ex-post impacts of improved cowpea varieties on productivity and net returns in Nigeria. J Agric Econ 71:165–183. https://doi.org/10.1111/1477-9552.12331
Manda J, Khonje MG, Alene AD et al (2020b) Does cooperative membership increase and accelerate agricultural technology adoption? Empirical evidence from Zambia. Technol Forecast Soc Change 158:120160. https://doi.org/10.1016/j.techfore.2020.120160
Marenya PP, Gebremariam G, Jaleta M, Rahut DB (2020) Sustainable intensification among smallholder maize farmers in Ethiopia: adoption and impacts under rainfall and unobserved heterogeneity. Food Policy 95:101941. https://doi.org/10.1016/j.foodpol.2020.101941
Martey E, Etwire PM, Kuwornu JKM (2020) Economic impacts of smallholder farmers’ adoption of drought-tolerant maize varieties. Land Use Policy 94:104524. https://doi.org/10.1016/j.landusepol.2020.104524
McFadden D (1973) Conditional logit analysis of qualitative choice behavior. Academic Press, New York
Google Scholar
Nakano Y, Tsusaka TW, Aida T, Pede VO (2018) Is farmer-to-farmer extension effective? The impact of training on technology adoption and rice farming productivity in Tanzania. World Dev 105:336–351. https://doi.org/10.1016/j.worlddev.2017.12.013
Ndiritu SW, Kassie M, Shiferaw B (2014) Are there systematic gender differences in the adoption of sustainable agricultural intensification practices? Evidence from Kenya. Food Policy 49:117–127. https://doi.org/10.1016/j.foodpol.2014.06.010
Nkegbe P, Shankar B (2014) Adoption intensity of soil and water conservation practices by smallholders: evidence from Northern Ghana. Bio-Based Appl Econ 3:159
Nonvide GMA (2021) Adoption of agricultural technologies among rice farmers in Benin. Rev Dev Econ Rode. https://doi.org/10.1111/rode.12802
Oduniyi OS, Chagwiza C (2021) Impact of adoption of sustainable land management practices on food security of smallholder farmers in Mpumalanga province of South Africa. GeoJournal. https://doi.org/10.1007/s10708-021-10497-0
Ogemah VK (2017) Sustainable agriculture: Developing a common understanding for modernization of agriculture in Africa. Afr J Food Agric Nutr Dev 17:11673–11690. https://doi.org/10.18697/ajfand.77.16560
Ojo TO, Ogundeji AA, Belle JA (2021) Climate change perception and impact of on-farm demonstration on intensity of adoption of adaptation strategies among smallholder farmers in South Africa. Technol Forecast Soc Change 172:121031. https://doi.org/10.1016/j.techfore.2021.121031
Onyeneke RU (2021) Does climate change adaptation lead to increased productivity of rice production? Lessons from Ebonyi State, Nigeria. Renew Agric Food Syst 36:54–68. https://doi.org/10.1017/S1742170519000486
Oparinde LO (2021) Fish farmers’ welfare and climate change adaptation strategies in southwest, Nigeria: application of multinomial endogenous switching regression model. Aquac Econ Manag 25:450–471. https://doi.org/10.1080/13657305.2021.1893863
Oyetunde Usman Z, Oluseyi Olagunju K, Rafiat Ogunpaimo O (2020) Determinants of adoption of multiple sustainable agricultural practices among smallholder farmers in Nigeria. Int Soil Water Conserv Res. https://doi.org/10.1016/j.iswcr.2020.10.007
Paudel GP, Gartaula H, Rahut DB, Craufurd P (2020) Gender differentiated small-scale farm mechanization in Nepal hills: an application of exogenous switching treatment regression. Technol Soc 61:101250. https://doi.org/10.1016/j.techsoc.2020.101250
Pham H, Chuah S, Feeny S (2021) Factors affecting the adoption of sustainable agricultural practices: findings from panel data for Vietnam. Ecol Econ 184:107000. https://doi.org/10.1016/j.ecolecon.2021.107000
Pizer SD (2016) Falsification testing of instrumental variables methods for comparative effectiveness research. Health Serv Res 51:790–811. https://doi.org/10.1111/1475-6773.12355
Rose DC, Sutherland WJ, Barnes AP et al (2019) Integrated farm management for sustainable agriculture: lessons for knowledge exchange and policy. Land Use Policy 81:834–842. https://doi.org/10.1016/j.landusepol.2018.11.001
Sarr M, Bezabih Ayele M, Kimani ME, Ruhinduka R (2021) Who benefits from climate-friendly agriculture? The marginal returns to a rainfed system of rice intensification in Tanzania. World Dev 138:105160. https://doi.org/10.1016/j.worlddev.2020.105160
Smale M, Assima A, Kergna A et al (2018) Farm family effects of adopting improved and hybrid sorghum seed in the Sudan Savanna of West Africa. Food Policy 74:162–171. https://doi.org/10.1016/j.foodpol.2018.01.001
Struik PC, Klerkx L, van Huis A, Röling NG (2014) Institutional change towards sustainable agriculture in West Africa. Int J Agric Sustain 12:203–213. https://doi.org/10.1080/14735903.2014.909641
Suvedi M, Ghimire R, Kaplowitz M (2017) Farmers’ participation in extension programs and technology adoption in rural Nepal: a logistic regression analysis. J Agric Educ Ext 23:351–371. https://doi.org/10.1080/1389224X.2017.1323653
Tambo JA, Matimelo M, Ndhlovu M et al (2021) Gender-differentiated impacts of plant clinics on maize productivity and food security: evidence from Zambia. World Dev 145:105519. https://doi.org/10.1016/j.worlddev.2021.105519
Teklewold H, Kassie M, Shiferaw B (2013a) Adoption of multiple sustainable agricultural practices in rural Ethiopia. J Agric Econ 64:597–623. https://doi.org/10.1111/1477-9552.12011
Teklewold H, Kassie M, Shiferaw B, Köhlin G (2013b) Cropping system diversification, conservation tillage and modern seed adoption in Ethiopia: impacts on household income, agrochemical use and demand for labor. Ecol Econ 93:85–93. https://doi.org/10.1016/j.ecolecon.2013.05.002
Thinda KT, Ogundeji AA, Belle JA, Ojo TO (2021) Determinants of relevant constraints inhibiting farmers’ adoption of climate change adaptation strategies in South Africa. J Asian Afr Stud 56:610–627. https://doi.org/10.1177/0021909620934836
Tinonin C, Azzarri C, Haile B et al (2016) Africa RISING Baseline Evaluation Survey (ARBES) report for Ghana
World Bank (2010) Economics of adaptation to climate change: Ghana country study. Washington, DC
Yang Q, Zhu Y, Liu L, Wang F (2022) Land tenure stability and adoption intensity of sustainable agricultural practices in banana production in China. J Clean Prod 338:130553. https://doi.org/10.1016/j.jclepro.2022.130553
Yu L, Chen C, Niu Z et al (2021) Risk aversion, cooperative membership and the adoption of green control techniques: Evidence from China. J Clean Prod 279:123288. https://doi.org/10.1016/j.jclepro.2020.123288
Zeweld W, Van Huylenbroeck G, Tesfay G et al (2020) Sustainable agricultural practices, environmental risk mitigation and livelihood improvements: empirical evidence from Northern Ethiopia. Land Use Policy 95:103799. https://doi.org/10.1016/j.landusepol.2019.01.002
Zhang S, Sun Z, Ma W, Valentinov V (2020) The effect of cooperative membership on agricultural technology adoption in Sichuan, China. China Econ Rev 62:101334. https://doi.org/10.1016/j.chieco.2019.101334
Zheng H, Ma W, Li G (2021) Learning from neighboring farmers: Does spatial dependence affect adoption of drought-tolerant wheat varieties in China? Can J Agric Econ Can D’agroeconomie 69:519–537. https://doi.org/10.1111/cjag.12294
Zhou X, Ma W, Li G (2018) Draft animals, farm machines and sustainable agricultural production: insight from China. Sustainability 10:3015. https://doi.org/10.3390/su10093015
Zhou X, Ma W, Renwick A, Li G (2020) Off-farm work decisions of farm couples and land transfer choices in rural China. Appl Econ 52:6229–6247. https://doi.org/10.1080/00036846.2020.1788709
Download references
Acknowledgements
The authors gratefully acknowledge the financial support from NZAID scholarship from MFAT and Lincoln university research fund. We want to thank IITA and IFPRI for making the data from the Africa RISING Project readily accessible. We also want to thank Dr. Gideon Danso-Abbeam for his helpful comments and suggestions.
No funding was received in the carrying out of this research.
Author information
Authors and affiliations.
Department of Global Value Chains and Trade, Faculty of Agribusiness and Commerce, Lincoln University, Christchurch, New Zealand
Edinam Dope Setsoafia, Wanglin Ma & Alan Renwick
You can also search for this author in PubMed Google Scholar
Contributions
All authors read and approved the final manuscript.
Corresponding author
Correspondence to Edinam Dope Setsoafia .
Ethics declarations
Competing interests.
The authors declare there is no conflict of interest.
Additional information
Publisher's note.
Springer Nature remains neutral with regard to jurisdictional claims in published maps and institutional affiliations.
Rights and permissions
Open Access This article is licensed under a Creative Commons Attribution 4.0 International License, which permits use, sharing, adaptation, distribution and reproduction in any medium or format, as long as you give appropriate credit to the original author(s) and the source, provide a link to the Creative Commons licence, and indicate if changes were made. The images or other third party material in this article are included in the article's Creative Commons licence, unless indicated otherwise in a credit line to the material. If material is not included in the article's Creative Commons licence and your intended use is not permitted by statutory regulation or exceeds the permitted use, you will need to obtain permission directly from the copyright holder. To view a copy of this licence, visit http://creativecommons.org/licenses/by/4.0/ .
Reprints and permissions
About this article
Cite this article.
Setsoafia, E.D., Ma, W. & Renwick, A. Effects of sustainable agricultural practices on farm income and food security in northern Ghana. Agric Econ 10 , 9 (2022). https://doi.org/10.1186/s40100-022-00216-9
Download citation
Received : 07 September 2021
Revised : 05 February 2022
Accepted : 04 March 2022
Published : 15 March 2022
DOI : https://doi.org/10.1186/s40100-022-00216-9
Share this article
Anyone you share the following link with will be able to read this content:
Sorry, a shareable link is not currently available for this article.
Provided by the Springer Nature SharedIt content-sharing initiative
- Sustainable agriculture practices
- Farm income
- Food security
JEL Classification
Loading metrics
Open Access
Climate change resilient agricultural practices: A learning experience from indigenous communities over India
Affiliation South Asian Forum for Environment, India
* E-mail: [email protected] , [email protected]
Affiliation Ecole Polytechnique Fédérale de Lausanne (Swiss Federal Institute of Technology), Lausanne, Switzerland

- Amitava Aich,
- Dipayan Dey,
- Arindam Roy
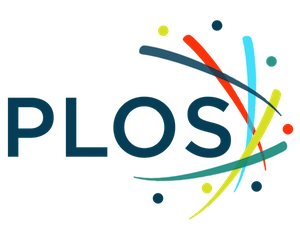
Published: July 28, 2022
- https://doi.org/10.1371/journal.pstr.0000022
- Reader Comments
The impact of climate change on agricultural practices is raising question marks on future food security of billions of people in tropical and subtropical regions. Recently introduced, climate-smart agriculture (CSA) techniques encourage the practices of sustainable agriculture, increasing adaptive capacity and resilience to shocks at multiple levels. However, it is extremely difficult to develop a single framework for climate change resilient agricultural practices for different agrarian production landscape. Agriculture accounts for nearly 30% of Indian gross domestic product (GDP) and provide livelihood of nearly two-thirds of the population of the country. Due to the major dependency on rain-fed irrigation, Indian agriculture is vulnerable to rainfall anomaly, pest invasion, and extreme climate events. Due to their close relationship with environment and resources, indigenous people are considered as one of the most vulnerable community affected by the changing climate. In the milieu of the climate emergency, multiple indigenous tribes from different agroecological zones over India have been selected in the present study to explore the adaptive potential of indigenous traditional knowledge (ITK)-based agricultural practices against climate change. The selected tribes are inhabitants of Eastern Himalaya (Apatani), Western Himalaya (Lahaulas), Eastern Ghat (Dongria-Gondh), and Western Ghat (Irular) representing rainforest, cold desert, moist upland, and rain shadow landscape, respectively. The effect of climate change over the respective regions was identified using different Intergovernmental Panel on Climate Change (IPCC) scenario, and agricultural practices resilient to climate change were quantified. Primary results indicated moderate to extreme susceptibility and preparedness of the tribes against climate change due to the exceptionally adaptive ITK-based agricultural practices. A brief policy has been prepared where knowledge exchange and technology transfer among the indigenous tribes have been suggested to achieve complete climate change resiliency.
Citation: Aich A, Dey D, Roy A (2022) Climate change resilient agricultural practices: A learning experience from indigenous communities over India. PLOS Sustain Transform 1(7): e0000022. https://doi.org/10.1371/journal.pstr.0000022
Editor: Ashwani Kumar, Dr. H.S. Gour Central University, INDIA
Copyright: © 2022 Aich et al. This is an open access article distributed under the terms of the Creative Commons Attribution License , which permits unrestricted use, distribution, and reproduction in any medium, provided the original author and source are credited.
Funding: The authors received no specific funding for this work.
Competing interests: The authors have declared that no competing interests exist.
1 Introduction
Traditional agricultural systems provide sustenance and livelihood to more than 1 billion people [ 1 – 3 ]. They often integrate soil, water, plant, and animal management at a landscape scale, creating mosaics of different land uses. These landscape mosaics, some of which have existed for hundreds of years, are maintained by local communities through practices based on traditional knowledge accumulated over generations [ 4 ]. Climate change threatens the livelihood of rural communities [ 5 ], often in combination with pressures coming from demographic change, insecure land tenure and resource rights, environmental degradation, market failures, inappropriate policies, and the erosion of local institutions [ 6 – 8 ]. Empowering local communities and combining farmers’ and external knowledge have been identified as some of the tools for meeting these challenges [ 9 ]. However, their experiences have received little attention in research and among policy makers [ 10 ].
Traditional agricultural landscapes as linked social–ecological systems (SESs), whose resilience is defined as consisting of 3 characteristics: the capacity to (i) absorb shocks and maintain function; (ii) self-organize; (iii) learn and adapt [ 11 ]. Resilience is not about an equilibrium of transformation and persistence. Instead, it explains how transformation and persistence work together, allowing living systems to assimilate disturbance, innovation, and change, while at the same time maintaining characteristic structures and processes [ 12 ]. Agriculture is one of the most sensitive systems influenced by changes in weather and climate patterns. In recent years, climate change impacts have been become the greatest threats to global food security [ 13 , 14 ]. Climate change results a decline in food production and consequently rising food prices [ 15 , 16 ]. Indigenous people are good observers of changes in weather and climate and acclimatize through several adaptive and mitigation strategies [ 17 , 18 ].
Traditional agroecosystems are receiving rising attention as sustainable alternatives to industrial farming [ 19 ]. They are getting increased considerations for biodiversity conservation and sustainable food production in changing climate [ 20 ]. Indigenous agriculture systems are diverse, adaptable, nature friendly, and productive [ 21 ]. Higher vegetation diversity in the form of crops and trees escalates the conversion of CO 2 to organic form and consequently reducing global warming [ 22 ]. Mixed cropping not only decreases the risk of crop failure, pest, and disease but also diversifies the food supply [ 23 ]. It is estimated that traditional multiple cropping systems provide 15% to 20% of the world’s food supply [ 1 ]. Agro-forestry, intercropping, crop rotation, cover cropping, traditional organic composting, and integrated crop-animal farming are prominent traditional agricultural practices [ 24 , 25 ].
Traditional agricultural landscapes refer to the landscapes with preserved traditional sustainable agricultural practices and conserved biodiversity [ 26 , 27 ]. They are appreciated for their aesthetic, natural, cultural, historical, and socioeconomic values [ 28 ]. Since the beginning of agriculture, peasants have been continually adjusting their agriculture practices with change in climatic conditions [ 29 ]. Indigenous farmers have a long history of climate change adaptation through making changes in agriculture practices [ 30 ]. Indigenous farmers use several techniques to reduce climate-driven crop failure such as use of drought-tolerant local varieties, polyculture, agro-forestry, water harvesting, and conserving soil [ 31 – 33 ]. Indigenous peasants use various natural indicators to forecast the weather patterns such as changes in the behavior of local flora and fauna [ 34 , 35 ].
The climate-smart agriculture (CSA) approach [ 36 ] has 3 objectives: (i) sustainably enhancing agricultural productivity to support equitable increase in income, food security, and development; (ii) increasing adaptive capacity and resilience to shocks at multiple levels, from farm to national; and (iii) reducing Green House Gases (GHG) emissions and increasing carbon sequestration where possible. Indigenous peoples, whose livelihood activities are most respectful of nature and the environment, suffer immediately, directly, and disproportionately from climate change and its consequences. Indigenous livelihood systems, which are closely linked to access to land and natural resources, are often vulnerable to environmental degradation and climate change, especially as many inhabit economically and politically marginal areas in fragile ecosystems in the countries likely to be worst affected by climate change [ 25 ]. The livelihood of many indigenous and local communities, in particular, will be adversely affected if climate and associated land-use change lead to losses in biodiversity. Indigenous peoples in Asia are particularly vulnerable to changing weather conditions resulting from climate change, including unprecedented strength of typhoons and cyclones and long droughts and prolonged floods [ 15 ]. Communities report worsening food and water insecurity, increases in water- and vector-borne diseases, pest invasion, destruction of traditional livelihoods of indigenous peoples, and cultural ethnocide or destruction of indigenous cultures that are linked with nature and agricultural cycles [ 37 ].
The Indian region is one of the world’s 8 centres of crop plant origin and diversity with 166 food/crop species and 320 wild relatives of crops have originated here (Dr R.S. Rana, personal communication). India has 700 recorded tribal groups with population of 104 million as per 2011 census [ 38 ] and many of them practicing diverse indigenous farming techniques to suit the needs of various respective ecoclimatic zones. The present study has been designed as a literature-based analytical review of such practices among 4 different ethnic groups in 4 different agroclimatic and geographical zones of India, viz, the Apatanis of Arunachal Pradesh, the Dongria Kondh of Niamgiri hills of Odisha, the Irular in the Nilgiris, and the Lahaulas of Himachal Pradesh to evaluating the following objectives: (i) exploring comparatively the various indigenous traditional knowledge (ITK)-based farming practices in the different agroclimatic regions; (ii) climate resiliency of those practices; and (iii) recommending policy guidelines.
2 Methodology
2.1 systematic review of literature.
An inventory of various publications in the last 30 years on the agro biodiversity, ethno botany, traditional knowledge, indigenous farming practices, and land use techniques of 4 different tribes of India in 4 different agroclimatic and geographical zones viz, the Apatanis of Arunachal Pradesh, the Dongria Kondh of Niamgiri hills of Odisha, the Irular in the Nilgiris, and the Lahaulas of Himachal Pradesh has been done based on key word topic searches in journal repositories like Google Scholar. A small but significant pool of led and pioneering works has been identified, category, or subtopics are developed most striking observations noted.
2.2 Understanding traditional practices and climate resiliency
The most striking traditional agricultural practices of the 4 major tribes were noted. A comparative analysis of different climate resilient traditional practices of the 4 types were made based on existing information available via literature survey. Effects of imminent dangers of possible extreme events and impact of climate change on these 4 tribes were estimated based on existing facts and figures. A heat map representing climate change resiliency of these indigenous tribes has been developed using R-programming language, and finally, a reshaping policy framework for technology transfers and knowledge sharing among the tribes for successfully helping them to achieve climate resiliency has been suggested.

2.3 Study area
Four different agroclimatic zones and 4 different indigenous groups were chosen for this particular study. The Apatanis live in the small plateau called Zero valley ( Fig 1 ) surrounded by forested mountains of Eastern Himalaya in the Lower Subansiri district of Arunachal Pradesh. It is located at 27.63° N, 93.83° E at an altitude ranging between 1,688 m to 2,438 m. Rainfall is heavy and can be up to 400 mm in monsoon months. Temperature varies from moderate in summer to very cold in the winter months. Their approximate population is around 12,806 (as per 2011 census), and Tibetan and Ahom sources indicate that they have been inhabiting the area from at least the 15th century and probably much earlier ( https://whc.unesco.org/en/tentativelists/5893/ ).
- PPT PowerPoint slide
- PNG larger image
- TIFF original image
The base map is prepared using QGIS software.
https://doi.org/10.1371/journal.pstr.0000022.g001
The Lahaulas are the inhabitants of Lahaul valley ( Fig 1 ) that is located in the western Himalayan region of Lahaul and Spiti and lies between the Pir Panjal in the south and Zanskar in the north. It is located between 76° 46′ and 78° 41′ east longitudes and between 31° 44′ and 32° 59′ north altitudes. The Lahaul valley receives scanty rainfalls, almost nil in summer, and its only source of moisture is snow during the winter. Temperature is generally cold. The combined population of Lahaul and Spiti is 31,564 (as per 2011 census).
The Dongria Kondh is one of the officially designated primitive tribal group (PTG) in the Eastern Ghat region of the state Orissa. They are the original inhabitants of Niyamgiri hilly region ( Fig 1 ) that extends to Rayagada, Koraput, and Kalahandi districts of south Orissa. Dongria Kondhs have an estimated population of about 10,000 and are distributed in around 120 settlements, all at an altitude up to 1,500 above the sea level [ 39 ]. It is located between 190 26′ to 190 43′ N latitude and 830 18′ to 830 28′ E longitudes with a maximum elevation of 1,516 meters. The Niyamgiri hill range abounds with streams. More than 100 streams flows from the Niyamgiri hills and 36 streams originate from Niyamgiri plateau (just below the Niyam Raja), and most of the streams are perennial. Niyamgiri hills have been receiving high rainfall since centuries and drought is unheard of in this area.
The Irular tribes inhabit the Palamalai hills and Nilgiris of Western Ghats ( Fig 1 ). Their total population may be 200,000 (as per 2011 census). The Palamali Hills is situated in the Salem district of Tamil Nadu, lies between 11° 14.46′ and 12° 53.30′ north latitude and between 77° 32.52′ to 78° 35.05′ east longitude. It is located 1,839 m from the mean sea level (MSL) and more over the climate of the district is whole dry except north east monsoon seasons [ 40 , 41 ]. Nilgiri district is hilly, lying at an elevation of 1,000 to 2,600 m above MSL and divided between the Nilgiri plateau and the lower, smaller Wayanad plateau. The district lies at the juncture of the Western Ghats and the Eastern Ghats. Its latitudinal and longitudinal location is 130 km (latitude: 11° 12 N to 11° 37 N) by 185 km (longitude 76° 30 E to 76° 55 E). It has cooler and wetter climate with high average rainfall.
3 Results and discussion
3.1 indigenous agricultural practices in 4 different agro-biodiversity hotspots.
Previous literatures on the agricultural practices of indigenous people in 4 distinct agro-biodiversity hotspots did not necessarily focus on climate resilient agriculture. The authors of these studies had elaborately discussed about the agro-biodiversity, farming techniques, current scenario, and economical sustainability in past and present context of socioecological paradigm. However, no studies have been found to address direct climate change resiliency of traditional indigenous agricultural practices over Indian subcontinent to the best of our knowledge. The following section will primarily focus on the agricultural practices of indigenous tribes and how they can be applied on current eco-agricultural scenario in the milieu of climate change over different agricultural macroenvironments in the world.
3.1.1 Apatani tribes (Eastern Himalaya).
The Apatanis practice both wet and terrace cultivation and paddy cum fish culture with finger millet on the bund (small dam). Due to these special attributes of sustainable farming systems and people’s traditional ecological knowledge in sustaining ecosystems, the plateau is in the process of declaring as World Heritage centre [ 42 – 44 ]. The Apatanis have developed age-old valley rice cultivation has often been counted to be one of the advanced tribal communities in the northeastern region of India [ 45 ]. It has been known for its rich economy for decades and has good knowledge of land, forest, and water management [ 46 ]. The wet rice fields are irrigated through well-managed canal systems [ 47 ]. It is managed by diverting numerous streams originated in the forest into single canal and through canal each agriculture field is connected with bamboo or pinewood pipe.
The entire cultivation procedure by the Apatani tribes are organic and devoid of artificial soil supplements. The paddy-cum-fish agroecosystem are positioned strategically to receive all the run off nutrients from the hills and in addition to that, regular appliance of livestock manure, agricultural waste, kitchen waste, and rice chaff help to maintain soil fertility [ 48 ]. Irrigation, cultivation, and harvesting of paddy-cum-fish agricultural system require cooperation, experience, contingency plans, and discipline work schedule. Apatani tribes have organized tasks like construction and maintenance of irrigation, fencing, footpath along the field, weeding, field preparation, transplantation, harvesting, and storing. They are done by the different groups of farmers and supervised by community leaders (Gaon Burha/Panchayat body). Scientific and place-based irrigation solution using locally produced materials, innovative paddy-cum-fish aquaculture, community participation in collective farming, and maintaining agro-biodiversity through regular usage of indigenous landraces have potentially distinguished the Apatani tribes in the context of agro-biodiversity regime on mountainous landscape.
3.1.2 Lahaula (Western Himalaya).
The Lahaul tribe has maintained a considerable agro-biodiversity and livestock altogether characterizing high level of germ plasm conservation [ 49 ]. Lahaulas living in the cold desert region of Lahaul valley are facultative farmers as they able to cultivate only for 6 months (June to November) as the region remained ice covered during the other 6 months of the year. Despite of the extreme weather conditions, Lahaulas are able to maintain high level of agro-biodiversity through ice-water harvesting, combinatorial cultivation of traditional and cash crops, and mixed agriculture–livestock practices. Indigenous practices for efficient use of water resources in such cold arid environment with steep slopes are distinctive. Earthen channels (Nullah or Kuhi) for tapping melting snow water are used for irrigation. Channel length run anywhere from a few meters to more than 5 km. Ridges and furrows transverse to the slope retard water flow and soil loss [ 50 ]. Leaching of soil nutrients due to the heavy snow cover gradually turns the fertile soil into unproductive one [ 51 ]. The requirement of high quantity organic manure is met through composting livestock manure, night soil, kitchen waste, and forest leaf litter in a specially designed community composting room. On the advent of summer, compost materials are taken into the field for improving the soil quality.
Domesticated Yaks ( Bos grunniens ) is crossed with local cows to produce cold tolerant offspring of several intermediate species like Gari, Laru, Bree, and Gee for drought power and sources of protein. Nitrogen fixing trees like Seabuckthrone ( Hippophae rhamnoides ) are also cultivated along with the crops to meet the fuels and fodder requires for the long winter period. Crop rotation is a common practice among the Lahaulas. Domesticated wild crop, local variety, and cash crops are rotated to ensure the soil fertility and maintaining the agro-biodiversity. Herbs and indigenous medicinal plants are cultivated simultaneously with food crops and cash crop to maximize the farm output. A combinatorial agro-forestry and agro-livestock approach of the Lahaulas have successfully able to generate sufficient revenue and food to sustain 6 months of snow-covered winter in the lap of western Himalayan high-altitude landscape. This also helps to maintain the local agro-biodiversity of the immensely important ecoregion.
3.1.3 Dongria Kondh (Eastern Ghat).
Dongria Kondh tribes, living at the semiarid hilly range of Eastern Ghats, have been applying sustainable agro-forestry techniques and a unique mixed crop system for several centuries since their establishment in the tropical dry deciduous hilly forest ecoregion. The forest is a source for 18 different non-timber forest products like mushroom, bamboo, fruits, vegetables, seeds, leaf, grass, and medicinal products. The Kondh people sustainably uses the forest natural capital such a way that maintain the natural stock and simultaneously ensure the constant flow of products. Around 70% of the resources have been consumed by the tribes, whereas 30% of the resources are being sold to generate revenue for further economic and agro-forest sustainability [ 52 ]. The tribe faces moderate to acute food grain crisis during the post-sowing monsoon period and they completely rely upon different alternative food products from the forest. The system has been running flawlessly until recent time due to the aggressive mining activity, natural resources depleted significantly, and the food security have been compromised [ 53 ].
However, the Kondh farmer have developed a very interesting agrarian technique where they simultaneously grow 80 varieties of different crops ranging from paddy, millet, leaves, pulses, tubers, vegetables, sorghum, legumes, maize, oil-seeds, etc. [ 54 ]. In order to grow so many crops in 1 dongor (the traditional farm lands of Dongria Kondhs on lower hill slopes), the sowing period and harvesting period extends up to 5 months from April till the end of August and from October to February basing upon climatic suitability, respectively.
Genomic profiling of millets like finger millet, pearl millet, and sorghum suggest that they are climate-smart grain crops ideal for environments prone to drought and extreme heat [ 55 ]. Even the traditional upland paddy varieties they use are less water consuming, so are resilient to drought-like conditions, and are harvested between 60 and 90 days of sowing. As a result, the possibility of complete failure of a staple food crop like millets and upland paddy grown in a dongor is very low even in drought-like conditions [ 56 ].
The entire agricultural method is extremely organic in nature and devoid of any chemical pesticide, which reduces the cost of farming and at the same time help to maintain environmental sustainability [ 57 ].
3.1.4 Irular tribes (Western Ghat).
Irulas or Irular tribes, inhabiting at the Palamalai mountainous region of Western Ghats and also Nilgiri hills are practicing 3 crucial age-old traditional agricultural techniques, i.e., indigenous pest management, traditional seed and food storage methods, and age-old experiences and thumb rules on weather prediction. Similar to the Kondh tribes, Irular tribes also practice mixed agriculture. Due to the high humidity in the region, the tribes have developed and rigorously practices storage distinct methods for crops, vegetables, and seeds. Eleven different techniques for preserving seeds and crops by the Irular tribes are recorded till now. They store pepper seeds by sun drying for 2 to 3 days and then store in the gunny bags over the platform made of bamboo sticks to avoid termite attack. Paddy grains are stored with locally grown aromatic herbs ( Vitex negundo and Pongamia pinnata ) leaves in a small mud-house. Millets are buried under the soil (painted with cow dung slurry) and can be stored up to 1 year. Their storage structure specially designed to allow aeration protect insect and rodent infestation [ 58 ]. Traditional knowledge of cross-breeding and selection helps the Irular enhancing the genetic potential of the crops and maintaining indigenous lines of drought resistant, pest tolerant, disease resistant sorghum, millet, and ragi [ 59 , 60 ].
Irular tribes are also good observer of nature and pass the traditional knowledge of weather phenomenon linked with biological activity or atmospheric condition. Irular use the behavioral fluctuation of dragonfly, termites, ants, and sheep to predict the possibility of rainfall. Atmospheric phenomenon like ring around the moon, rainbow in the evening, and morning cloudiness are considered as positive indicator of rainfall, whereas dense fog is considered as negative indicator. The Irular tribes also possess and practice traditional knowledge on climate, weather, forecasting, and rainfall prediction [ 58 ]. The Irular tribes also gained extensive knowledge in pest management as 16 different plant-based pesticides have been documented that are all completely biological in nature. The mode of actions of these indigenous pesticides includes anti-repellent, anti-feedent, stomach poison, growth inhibitor, and contact poisoning. All of these pesticides are prepared from common Indian plants extract like neem, chili, tobacco, babul, etc.
The weather prediction thumb rules are not being validated with real measurement till now but understanding of the effect of forecasting in regional weather and climate pattern in agricultural practices along with biological pest control practices and seed conservation have made Irular tribe unique in the context of global agro-biodiversity conservation.
3.2 Climate change risk in indigenous agricultural landscape
The effect of climate change over the argo-ecological landscape of Lahaul valley indicates high temperature stress as increment of number of warm days, 0.16°C average temperature and 1.1 to 2.5°C maximum temperature are observed in last decades [ 61 , 62 ]. Decreasing trend of rainfall during monsoon and increasing trend of consecutive dry days in last several decades strongly suggest future water stress in the abovementioned region over western Himalaya. Studies on the western Himalayan region suggest presence of climate anomaly like retraction of glaciers, decreasing number of snowfall days, increasing incident of pest attack, and extreme events on western Himalayan region [ 63 – 65 ].
Apatani tribes in eastern Himalayan landscape are also experiencing warmer weather with 0.2°C increment in maximum and minimum temperature [ 66 ]. Although no significant trend in rainfall amount has been observed, however 11% decrease in rainy day and 5% to 15% decrease in rainfall amount by 2030 was speculated using regional climate model [ 67 ]. Increasing frequency of extreme weather events like flashfloods, cloudburst, landslide, etc. and pathogen attack in agricultural field will affect the sustainable agro-forest landscape of Apatani tribes. Similar to the Apatani and Lahaulas tribes, Irular and Dongria Kondh tribes are also facing climate change effect via increase in maximum and minimum temperature and decrease in rainfall and increasing possibility of extreme weather event [ 68 , 69 ]. In addition, the increasing number of forest fire events in the region is also an emerging problem due to the dryer climate [ 70 ].
Higher atmospheric and soil temperature in the crop growing season have direct impact on plant physiological processes and therefore has a declining effect on crop productivity, seedling mortality, and pollen viability [ 71 ]. Anomaly in precipitation amount and pattern also affect crop development by reducing plant growth [ 72 ]. Extreme events like drought and flood could alter soil fertility, reduce water holding capacity, increase nutrient run off, and negatively impact seed and crop production [ 73 ]. Agricultural pest attack increases at higher temperature as it elevates their food consumption capability and reproduction rate [ 74 ].
3.3 Climate resiliency through indigenous agro-forestry
Three major climate-resilient and environmentally friendly approaches in all 4 tribes can broadly classified as (i) organic farming; (ii) soil and water conservation and community farming; and (iii) maintain local agro-biodiversity. The practices under these 3 regimes have been listed in Table 1 .
https://doi.org/10.1371/journal.pstr.0000022.t001
Human and animal excreta, plant residue, ashes, decomposed straw, husk, and other by-products are used to make organic fertilizer and compost material that helps to maintain soil fertility in the extreme orographic landscape with high run-off. Community farming begins with division of labour and have produced different highly specialized skilled individual expert in different farming techniques. It needs to be remembered that studied tribes live in an area with complex topological feature and far from advance technological/logistical support. Farming in such region is extremely labour intensive, and therefore, community farming has become essential for surviving. All 4 tribes have maintained their indigenous land races of different crops, cereal, vegetables, millets, oil-seeds, etc. that give rises to very high agro-biodiversity in all 4 regions. For example, Apatanis cultivate 106 species of plants with 16 landraces of indigenous rice and 4 landraces of indigenous millet [ 75 ]. Similarly, 24 different crops, vegetables, and medicinal plants are cultivated by the Lahaulas, and 50 different indigenous landraces are cultivated by Irular and Dongria Kondh tribes.
The combination of organic firming and high indigenous agro-biodiversity create a perfect opportunity for biological control of pests. Therefore, other than Irular tribe, all 3 tribes depend upon natural predator like birds and spiders, feeding on the indigenous crop, for predation of pests. Irular tribes developed multiple organic pest management methods from extract of different common Indian plants. Apatani and Lahaulas incorporate fish and livestock into their agricultural practices, respectively, to create a circular approach to maximize the utilization of waste material produced. At a complex topographic high-altitude landscape where nutrient run-off is very high, the practices of growing plants with animals also help to maintain soil fertility. Four major stresses due to the advancement of climate change have been identified in previous section, and climate change resiliency against these stresses has been graphically presented in Fig 2 .
https://doi.org/10.1371/journal.pstr.0000022.g002
Retraction of the glaciers and direct physiological impact on the livestock due to the temperature stress have made the agricultural practices of the Lahaula’s vulnerable to climate change. However, Irular and Dongria Kondh tribes are resilient to the temperature stress due to their heat-resistant local agricultural landraces, and Apatanis will remain unaffected due to their temperate climate and vast forest cover. Dongria Kondh tribe will successfully tackle the water stress due to their low-water farming techniques and simultaneous cultivation of multiple crops that help to retain the soil moisture by reducing evaporation. Hundreds of perennial streams of Nyamgiri hills are also sustainably maintained and utilised by the Dongria Kondhs along with the forests, which gives them enough subsistence in form of non-timber forest products (NTFPs). However, although Apatani and Lahuala tribe extensively reuse and recirculate water in their field but due to the higher water requirement of paddy-cum-fish and paddy-cum-livestock agriculture, resiliency would be little less compared to Dongria Kondh.
Presence of vast forest cover, very well-structured irrigation system, contour agriculture and layered agricultural field have provided resiliency to the Apatani’s from extreme events like flash flood, landslides, and cloud burst. Due to their seed protection practices and weather prediction abilities, Irular tribe also show resiliency to the extreme events. However, forest fire and flash flood risk in both Eastern Ghat and Western Ghat have been increased and vegetation has significantly decreased in recent past. High risk of flash flood, land slide, avalanches, and very low vegetation coverage have made the Lahaulas extremely vulnerable to extreme events. Robust pest control methods of Irular tribe and age-old practices of intercropping, mixed cropping, and sequence cropping of the Dongria Kondh tribe will resist pest attack in near future.
3.4 Reshaping policy
Temperature stress, water stress, alien pest attack, and increasing risk of extreme events are pointed out as the major risks in the above described 4 indigenous tribes. However, every tribe has shown their own climate resiliency in their traditional agrarian practices, and therefore, a technology transfers and knowledge sharing among the tribes would successfully help to achieve the climate resilient closure. The policy outcome may be summarizing as follows:
- Designing, structuring and monitoring of infrastructural network of Apatani and Lahaul tribes (made by bamboo in case of Apatanis and Pine wood and stones in case of Lahaulas) for waster harvesting should be more rugged and durable to resilient against increasing risk of flash flood and cloud burst events.
- Water recycling techniques like bunds, ridges, and furrow used by Apatani and Lahaul tribes could be adopted by Irular and Dongria Kondh tribes as Nilgiri and Koraput region will face extreme water stress in coming decades.
- Simultaneous cultivation of multiple crops by the Dongria Kondh tribe could be acclimated by the other 3 tribes as this practice is not only drought resistance but also able to maximize the food security of the population.
- Germplasm storage and organic pest management knowledge by the Irular tribes could be transferred to the other 3 tribes to tackle the post-extreme event situations and alien pest attack, respectively.
- Overall, it is strongly recommended that the indigenous knowledge of agricultural practices needs to be conserved. Government and educational institutions need to focus on harvesting the traditional knowledge by the indigenous community.
3.5 Limitation
One of the major limitations of the study is lack of significant number of quantifiable literature/research articles about indigenous agricultural practices over Indian subcontinent. No direct study assessing risk of climate change among the targeted agroecological landscapes has been found to the best of our knowledge. Therefore, the current study integrates socioeconomic status of indigenous agrarian sustainability and probable climate change risk in the present milieu of climate emergency of 21st century. Uncertainty in the current climate models and the spatiotemporal resolution of its output is also a minor limitation as the study theoretically correlate and proposed reshaped policy by using the current and future modeled agro-meteorological parameters.
4. Conclusions
In the present study, an in-depth analysis of CSA practices among the 4 indigenous tribes spanning across different agro-biodiversity hotspots over India was done, and it was observed that every indigenous community is more or less resilient to the adverse effect of climate change on agriculture. Thousands years of traditional knowledge has helped to develop a unique resistance against climate change among the tribes. However, the practices are not well explored through the eyes of modern scientific perspective, and therefore, might goes extinct through the course of time. A country-wide study on the existing indigenous CSA practices is extremely important to produce a database and implementation framework that will successfully help to resist the climate change effect on agrarian economy of tropical countries. Perhaps the most relevant aspect of the study is the realization that economically and socially backward farmers cope with and even prepare for climate change by minimizing crop failure through increased use of drought tolerant local varieties, water harvesting, mixed cropping, agro-forestry, soil conservation practices, and a series of other traditional techniques.
- View Article
- Google Scholar
- 2. Nori M, Switzer J, Crawford A. Herding on the brink: towards a global survey of pastoral communities and conflict. An Occasional Working Paper from the International Union for Conservation of Nature (IUCN) Commission on Environmental. Economic and Social Policy. Gland: IUCN; 2005.
- 3. Howard P, Puri R, Smith L. Globally important agricultural heritage systems: a scientific conceptual framework and strategic principles. Rome: FAO; 2009.
- 6. Adger WN, Brooks N, Bentham G, Agnew M, Eriksen S. New indicators of vulnerability and adaptive capacity. Norwich: Tyndall Centre for Climate Change Research; 2005.
- PubMed/NCBI
- 9. IAASTD (International Assessment of Agricultural Knowledge, Science and Technology for Development). Agriculture at a crossroads, international assessment of agricultural knowledge, science and technology for development global report. Washington, DC: Island Press; 2009.
- 10. Salick J, Byg A. Indigenous peoples and climate change. Report of Symposium, 12–13 April 2007. University of Oxford and Missouri Botanical Garden. Oxford: Tyndall Centre Publication; 2007.
- 12. Westley F, Zimmerman B, Patton M. Getting to maybe. Toronto, Ontario, Canada: Random House of Canada; 2006.
- 25. PAR (Platform for Agrobiodiversity Research). Workshop report: experiences, knowledge gaps and opportunities for collaboration. The use of agrobiodiversity by indigenous peoples and rural communities in adapting to climate change [online]. Rome: Platform for Agrobiodiversity Research. 2009. Available from: https://satoyama-initiative.org/case_studies/the-use-of-agrobiodiversity-by-indigenous-and-traditional-agricultural-communities-in-adapting-to-climate-change/ PAR Chiang Mai Technical Report.doc [cited 2011 May 11].
- 32. Browder JO. Fragile lands in Latin America: strategies for sustainable development. Boulder: Westview Press; 1989.
- 36. FAO. “Climate-smart” agriculture: policies, practices and financing for food security, adaptation and mitigation. Rome. 2010.
- 45. Haimendorf CVF. The Apatanis and their neighbours. London: Oxford University Press; 1962.
- 65. Krishnan R, Shrestha AB, Ren G, Rajbhandari R, Saeed S, Sanjay J, et al. Unravelling climate change in the Hindu Kush Himalaya: rapid warming in the mountains and increasing extremes. In: The Hindu Kush Himalaya Assessment. Cham: Springer; 2019. p. 57–97.
- 69. TNSAPCC (Tamil Nadu State Action Plan for Climate Change reports). 2013. Available from: https://cag.gov.in/uploads/media/tamil-nadu-climate-change-action-plan-20200726073516.pdf .
Thank you for visiting nature.com. You are using a browser version with limited support for CSS. To obtain the best experience, we recommend you use a more up to date browser (or turn off compatibility mode in Internet Explorer). In the meantime, to ensure continued support, we are displaying the site without styles and JavaScript.
- View all journals
- Explore content
- About the journal
- Publish with us
- Sign up for alerts
- Published: 15 October 2018
Sustainable agriculture
Nature Sustainability volume 1 , page 531 ( 2018 ) Cite this article
6617 Accesses
4 Citations
2 Altmetric
Metrics details
- Agriculture
- Environmental impact
- Psychology and behaviour
- Sustainability
Achieving food security is possible, if we better understand the complexity of the agricultural system and re-design practices accordingly.
Early in September, the State of Food Security and Nutrition in the World report was released through a joint press conference at the FAO headquarters in Rome. The analysis ( The State of Food Security and Nutrition in the World FAO; 2018) is the outcome of a close collaboration between five United Nations agencies — the Food and Agriculture Organization, the International Fund for Agricultural Development, the United Nations Children’s Fund (UNICEF), the World Food Programme and the World Health Organization — and one of the key messages from the report is, perhaps not surprisingly, alarming: the world is not on track to meet the ‘Zero Hunger’ Sustainable Development Goal, SDG 2.
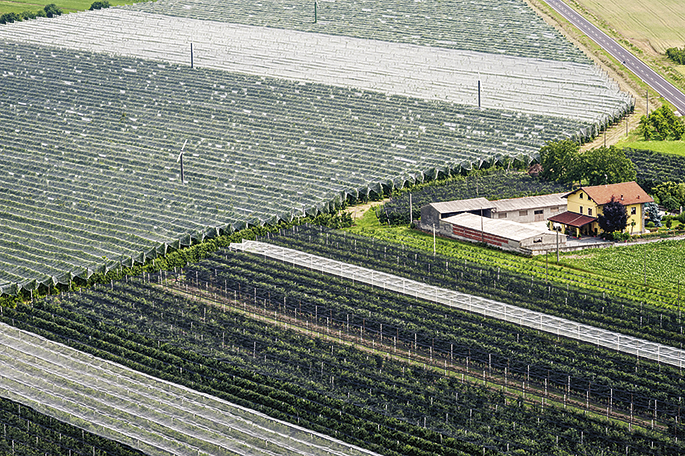
Alex Ramsay/Alamy Stock Photo
Drawing attention to the drivers of hunger and malnutrition, the report includes updated estimates of a number of indicators related to food security and its health implications, including the number of hungry people in the world, data on child stunting, adult obesity, and childhood obesity among others.
The report, which uses data from 2017, shows how hunger has increased worldwide for the third consecutive year, and childhood malnutrition has not improved or, even worse, in some cases has declined. Countries must take urgent action to meet SDG 2 by 2030.
This year’s edition of the annual report focuses on the need to build climate resilience for food security and nutrition. Acknowledging the dependency of our nutritional needs on the natural environment should indeed be a critical component of any food-policy strategy.
More specifically, the sustainability of the food system should be at the heart of the international food-security debate. Despite increasing hunger globally, the demand of food has been rising rapidly and has had a significant environmental cost: degradation of agricultural land, pollution of rivers and aquifers due to agro-chemicals, increased freshwater consumption, greenhouse-gas emissions from agriculture and land-use change, loss of agro-biodiversity and other negative consequences. All of these environmental impacts severely undermine our ability to continue to feed a growing population and ultimately will jeopardize the opportunity to meet SDG 2, unless more-sustainable food-production practices are embraced globally.
Enhanced agricultural productivity (intensification) has been a major response to the growing food demand, but intensification could be done better. In an Analysis by Pretty et al. published in our August issue, the authors underline how environmental considerations in agriculture intensification have been traditionally limited to minimizing negative impacts. Instead, with their analyses, they show that, for example, a move away from fertilizers to nitrogen-fixing legumes as part of rotations or intercropping could improve intensification without increasing environmental stress. Their point is that agriculture intensification can be sustainable if the system is adequately re-designed and if all players involved accept that no new designed system will succeed forever.
Increasing food production can impact conservation strategies — another issue likely to have long-term negative consequences for our ability to provide healthy levels of nutrition to all. Keesing and colleagues, in an Article in this issue, show however that a conflict between the two shouldn’t be the case. They analyse the potential trade-offs between management for wildlife and for livestock in an East African savannah, and find potential ecological and economic benefits from integrating the two.
However, even while considering effective strategies, it remains clear that human activities do affect biodiversity around the world, and that applies to agricultural practices as much as to other activities. In a Brief Communication in the August issue, Mehrabi et al. analyse the implications of the conservationists’ proposal to give back half the Earth’s surface to nature (the ‘Half-Earth’ project). Among other results, they find that, depending on the landscape conservation strategy, 23–25% of non-food calories and 3–29% of food calories from crops globally could be lost if the proposal were implemented. They do show that the trade-offs between agriculture and the Half-Earth proposal will be much lower if landscapes remain mosaics of shared land uses.
So, what are we left with? We can certainly improve agricultural practices as discussed earlier in this Editorial, and increase their sustainability. Will it be enough to achieve our societal goals? Perhaps we need to also look at our individual behaviour. We know that we need to manage our dependence on nature sustainably. And sustainable management hinges on deep understanding of human–nature relationships. Behavioural sciences can bring invaluable insights to our ways of mapping the complexity of such relationships. Going back to the sustainability of agricultural practices, the different ways in which individuals’ mindsets represent a system and the causal relationships among its components (mental models) can capture more or less complexity, as discussed in an Article by Levy and colleagues in our August issue. The authors show that, for example, mental models characterized by direct, unidirectional causation allow fast decision-making but might fail to anticipate consequences of actions in the presence of strong interdependences. Understanding these cognitive mechanisms has important implications for individual and collective decision-making about sustainable agriculture. And that is crucial to better orient food-security strategies. With the right set of changes in practices and interventions, informed by academic research and practice, there is still hope to achieve SDG 2 on time.
Rights and permissions
Reprints and permissions
About this article
Cite this article.
Sustainable agriculture. Nat Sustain 1 , 531 (2018). https://doi.org/10.1038/s41893-018-0163-4
Download citation
Published : 15 October 2018
Issue Date : October 2018
DOI : https://doi.org/10.1038/s41893-018-0163-4
Share this article
Anyone you share the following link with will be able to read this content:
Sorry, a shareable link is not currently available for this article.
Provided by the Springer Nature SharedIt content-sharing initiative
Quick links
- Explore articles by subject
- Guide to authors
- Editorial policies
Sign up for the Nature Briefing: Anthropocene newsletter — what matters in anthropocene research, free to your inbox weekly.

- Reference Manager
- Simple TEXT file
People also looked at
Review article, big data analysis for sustainable agriculture on a geospatial cloud framework.

- 1 Soil Management and Sugar Beet Research Unit, Agricultural Research Service, United States Department of Agriculture (USDA-ARS), Fort Collins, CO, United States
- 2 Environmental Systems Research Institute, National Government Unit, Redlands, CA, United States
- 3 Sustainable Agricultural Systems Laboratory, Agricultural Research Service, United States Department of Agriculture (USDA-ARS), Beltsville, MD, United States
- 4 Center for Agricultural Resources Research, Agricultural Research Service, United States Department of Agriculture (USDA-ARS), Fort Collins, CO, United States
Humanity is confronted with the grand challenge of how to increase agricultural production to achieve food security during the 21st century and feed a population that is expected to grow to 10 billion people. This needs to be done while maintaining sustainable agricultural systems and simultaneously facing challenges such as a changing climate, depletion of water resources, and the potential for increased erosion and loss of productivity due to the occurrence of extreme weather events. Precision Agriculture emerged out of the advances in the 1980s because of the development of several key technologies like GPS and satellite imagery. This paper argues that with the increasing impact of climate change, the next revolution in precision agriculture and agriculture in general will be driven by Sustainable Precision Agriculture and Environment (SPAE, similar to the 7 Rs), which could leverage past technologies combined with Big Data analysis. This new, technology-focused SPAE transitions from a site-specific management focus to the notion of global sustainability. To accomplish this transition, we introduced the WebGIS framework as an organizing principle that connects local, site-specific data generators called smart farms to a regional and global view of agriculture that can support both the agricultural industry and policymakers in government. This will help integrate databases located in networks of networks into a system of systems to achieve the needed SPAE management and connect field, watershed, national, and worldwide sustainability. Automation and the use of artificial intelligence (AI), internet of things (IoT), drones, robots, and Big Data serve as a basis for a global “Digital Twin,” which will contribute to the development of site-specific conservation and management practices that will increase incomes and global sustainability of agricultural systems.
Introduction
The 21st century presents formidable challenges to sustainability that humanity will have to confront. The need to increase agricultural production to ensure food security for a global population estimated to grow to 9–10 billion people in the coming decades while confronting a changing climate that threatens sustainability will put pressure on agricultural systems. The United Nations Secretary-General recently warned the global community that climatic changes are occurring at a faster rate than humanity is addressing them and that humanity will be impacted by sea level rise and more extreme weather ( United Nations, 2018 ). Recent reports released by the UN Intergovernmental Panel on Climate Change support these statements ( United Nations, 2018 ). The increased occurrence of extreme weather events will increase the potential for erosion in agricultural systems ( Pruski and Nearing, 2002 ; SWCS, 2003 ). Pruski and Nearing (2002) reported that erosion rates could increase by 1.7% for every 1% increase in total rainfall due to climate change. Without conservation practices humanity will not be able to adapt to a changing climate, as conservation practices will be key tools to maintain and increase the productivity and sustainability of agricultural systems ( Delgado et al., 2011 ; Walthall et al., 2012 ; Spiegal et al., 2018 ). Big data analysis will also be one of the key tools that will contribute to development of sustainable systems.
It is thought that crop production must be increased by 60–100% by the year 2050 to meet the nutritional needs of a future human population of 9–10 billion. Crop production systems that yield more food of higher nutritional content are needed, yet at the same time, they must have a diminished impact on the environment. Agricultural intensification during the 20th century was through the substantial use of fertilizer, pesticides, and irrigation, all at a significant environmental cost. These technologies were part of the Green Revolution that helped achieve food security for billions of people. However, the challenges of the 21st century are different, and soil and water conservation will be key to achieve food security, and sustainable precision agriculture and environment (SPAE) will be needed so that intensive agriculture and a changing climate will not generate additional impacts that could contribute to accelerating the pace of a changing climate. As a part of sustainable agriculture, next-generation cropping systems that couple biologically-based technologies (plant-beneficial microbes, cover crops) and precision agriculture (PA) and precision conservation (PC) need to be developed to decrease fertilizer, pesticide, and water inputs while increasing conservation effectiveness to maintain sustainable agriculture at a field level and sustainability across a watershed. Crop cultivars with enhanced nutritional content and enhanced tolerance to abiotic (drought, salinity, heat, etc.) and/or biotic (disease) stresses need to be developed using advanced breeding and biotechnology approaches. These enhanced cultivars will no doubt disrupt the status quo of agricultural business.
Central to SPAE and the rapid development of these cropping systems is the use of PA and PC in the development and use of technology with the capacity to manage and disseminate accurate data and information at all levels of the agricultural ecosystem. PA ( Pierce and Nowak, 1999 ), PC ( Berry et al., 2003 , 2005 ; Delgado and Berry, 2008 ; Sassenrath and Delgado, 2018 ) ( Figure 1 ), and sustainable agriculture “are inextricably linked” ( Berry et al., 2003 , 2005 ; Bongiovanni and Lowenberg-DeBoer, 2004 ). Sustainable agriculture and PC focus on increasing conservation effectiveness and stress environmental impact and sustainability, while PA is often about immediate cost savings at a specific location by optimizing returns on inputs. This paper focuses on sustainability at both a site-specific management scale and a global scale. For that reason, the emphasis is on information systems and their ability to support a variety of characteristics of PA, PC, and global sustainability.
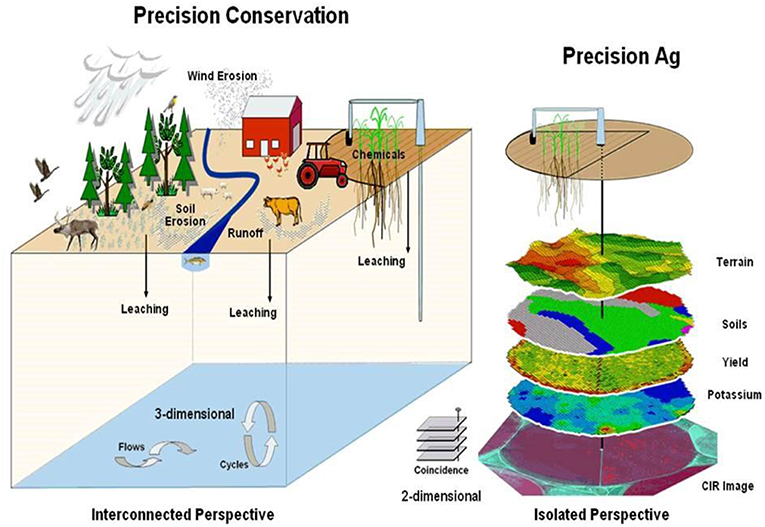
Figure 1 . The site-specific approach can be expanded to a three- dimensional scale approach that assesses inflows and outflows from fields to watershed and regional scales [Permission granted by Soil Water Conservation Society for reprint from source: ( Berry et al., 2003 )].
Inherent in both the complexity and accuracy of SPAE is the need to manage data spatially, which has traditionally been the realm of Geographic Information Systems (GIS) 1 For example, PA and PC use geospatial data and sensors for crop yield and any other measurable variable to apply the correct rate of fertilizer, water, and pesticide; manage drainage and water runoff; reduce movement of agrochemicals; and use the right management practices at the field and off-site ( Delgado et al., 2018a ). Both PA and PC allow the farmer to treat the production field as the heterogeneous surface it is (fertility, water, plant pathogens, slope, surface runoff, drainage, etc., which are all highly variable throughout the field) instead of as a homogeneous surface as it was treated in the past. SPAE also manages geospatial data, but its spatial relationships can be more abstract from the soil level to the molecular level in order to model more complex biological systems. In fact, Esri refers to this concept as the “science of where,” implying that GIS is evolving beyond the traditional geospatial realm of maps, images, etc. to modeling more complex relationships ( Dolan et al., 2006 ).
With increased adoption of PA and PC by farmers will come increased development and marketing of tools for PA and PC to speed up the adoption of technology. In the developed world primarily, advanced sensors and systems that deliver decision support tools directly to the farmer will be developed, allowing real-time decisions on the delivery of appropriate rate of inputs (water, fertilizer, pesticide) ( Fulton and Darr, 2018 ) as the farmer drives the tractor through the field, implements drainage systems ( Shedekar and Brown, 2018 ) and/or tries to simultaneously conserve wildlife ( McConnell and Burger, 2018 ).
The need for PA and PC will only heighten, as, for example, nutrient losses to the environment impact groundwater and surface water resources, such as has occurred with the hypoxic zone in the Gulf of Mexico ( Goolsby et al., 2001 ; Rabalais et al., 2002a , b ) and the hypoxic zone and microcystin levels in Lake Erie ( Smith et al., 2018 ). The World Health Organization (2011) has reported that microcystin concentrations above 1.0 μg L −1 in treated drinking water is not safe and is unhealthy for human consumption and in the USA, the EPA reports that for children less than 6 years of age the safe level is 0.3 μg L−1 ( United States Environmental Protection Agency, 2015 ). Water issues will continue to increase in many parts of the world in the near term, especially if there are legacy effects. New cropping systems with improved management practices must be developed that contribute to environmental sustainability to minimize negative impacts on air, soil and water. In addition, advanced crop cultivars must be developed that better use soil nutrient and water resources, are more resistant to environmental stress (temperature extremes, drought, flood, plant pathogens), and are packed with nutrients that are found deficient in populations in the developing world ( Bouis and Saltzman, 2017 ). This all must be done very rapidly as 30 years is not a long time when dealing with science and adoption of scientific technology. In other words, SPAE, which aims to preserve ecosystem services, must work with modern technologies and practices quickly through the rapid transfer of knowledge from the agricultural lab to the producer.
Over the past several decades, Information Technology (IT) has been the disruptive force in industries by driving out market inefficiencies through automation and better decision support tools that require the inclusion of both the citizens and consumers in the process. Like all industries, agriculture has not been immune to the constant disruptions over the past century (e.g., introduction of the tractor, PA, PC, and new governance models for dealing with inelasticity of demand, etc.). However, recent advances in computing infrastructure, sensor technology, big data, and advanced algorithms (e.g., Deep Learning) suggest that a major disruption or paradigm shift is on the horizon, leading to opportunities for SPAE entering the mainstream in a smart, advanced system for SPAE. IT also holds the key for accelerating knowledge transfer from the lab to the producer.
Eventually, a system based on these new technologies will be needed for mass transfer of genomic and other genetic data for the development of these advanced crop cultivars, for the management of agronomic data, and for the development of these next-generation production systems. Data generated from these cropping systems are inherently geospatial in that crop types and their environmental hardiness obviously vary regionally by latitude. Agricultural field crop production inputs and conservation management to achieve sustainable systems can vary considerably over space and time ( Pierce and Nowak, 1999 ; Berry et al., 2003 , 2005 ; Delgado and Berry, 2008 ; Gebbers and Adamchuk, 2010 ). Therefore, monitoring crop and environmental performance will highly depend not only on traditional methods of Earth Observation (EO), but also data generated in situ (i.e., ground truth).
Hence, geospatial solutions based on imagery from EO at all scales integrated with sensor networks will increasingly become critical for the operation of PA systems where resource inputs are applied at precise geo-specific field locations based on crop need. Solutions will eventually be needed to allow immediate feedback from digital farm communities regarding the performance of these new cropping systems; speeding their development based on immediate feedback to the labs and other interested parties. To accomplish this, we describe a “system of systems” approach to building a scientific network that integrates the scientific and farming communities, based on a common, global IT platform (i.e., the cloud).
Historical Challenges in Agricultural Technology Adoption for PA
Although PA has been around since the 1980s, the adoption of the technology has been slow. Schimmelphfenning (2016) reports that PA technology, for example, was used on about 30–50% of U.S. corn and soybean acres in 2012. Van der Wal (2019) suggests that the reason for poor adoption is due to the “growing complexity of adoption in the use of information technology” and the fact that “incomes in agriculture are generally low and young generations seek their prosperity in cities,” implying that those with technical prowess are heading to the cities for hi-tech jobs that pay better. PA also tends to be expensive ( Shama, 2017 ), which is reflected in the fact that it is mostly implemented on larger farms ( Schimmelphfenning, 2016 ) which can afford the complex and changing technology. However, low-tech precision agriculture/precision conservation approaches have been implemented by farmers in Sub-Saharan Africa, contributing to improved yields, incomes and conservation ( Jenrich, 2011 ). There are numerous reasons for the low adoption rates and the next few sections offer several common explanations. However, there are opportunities for leveraging existing technological trends.
Poor Adoption of Decision Support Tools
While the agricultural sector has a long tradition of relying on best practices rooted in science, the industry hasn't always been an early adopter of decision support tools or extension services that resulted in speeding up the adoption of new precision agricultural practices ( Rose et al., 2016 ). Ribaudo et al. (2011a) reported in a national study that 65 percent of the cropland studied (109 million acres) needed best practices for nitrogen management. There are several reasons for limited adoption including culture in the producer community, skills, current information management processes, etc. Perhaps the biggest reason, until recently, has been the limitations of both the technologies and agricultural systems models used to support PA, much less PC and SPAE ( Antle et al., 2017 ). Specifically, Antle et al. (2017) states that “many advances in data, information and communication technology of the past decade have not been fully exploited… [because of the] underinvestment in agricultural research, particularly in non-proprietary public good research, and in research aiming to improve the well-being of poor, smallholder farm households in the developing world.” While user-centered design techniques in the IT industry will improve adoption, an active area of research exists to more quantitatively understand positive adoption of new practices ( Kuehne et al., 2017 ).
That being said, technology adoption challenges in the IT industry are well understood and noted by Moore (1991) who discusses the diffusion of innovation through groups of technologists, early adopters who see the possibilities of innovation, pragmatists who resist change until an economic benefit is defined, the late majority adopters who require low risk and high reliability, and the laggards. By utilizing targeted messaging, Silicon Valley and other tech centers' success over the past few decades in disrupting markets is often attributed to the model cited in Moore (1991) , which ironically had its roots in the study of farming practice adoption ( Beal and Bohlen, 1957 ).
Limitations of Earth Observation Data in Agriculture
From a practical point of view, these historical technical limitations in the past have ranged from lack of standards, non-scalable systems, cost of sensors, and limited support from governments around the use of EO in agriculture or remotely sensed data that focused on climate change observations as opposed to agriculture. Although there was early adoption in agriculture in the US through programs like AgRISTARS and LACIE in the 1970s/80s ( Pinter et al., 2003 ), the Landsat series, for example, primarily focused on moderate spatial resolution (i.e., 30 × 30 m), which while good for crop monitoring at the macro level, was too course for PA. Luccio (2014) suggests that “…farm management decisions, such as weed detection and management, require imagery with a spatial resolution in the order of centimeters and, for emergent situations (such as to monitor nutrient stress and disease), a temporal resolution of <24 h.”
Likewise, these moderate resolution satellites, which were primarily designed for answering scientific questions, haven't always focused on the appropriate spectral bands (e.g., red edge band) for agriculture or frequency of data acquisition to make it easy to monitor crop growth during the growing season. Yet, because of the global coverage, these satellite networks have been recognized as the original generators of big data and represented a compromise or trade-off between spatial resolution and storage capacity. In recent years, commercial satellites, such as those from DigitalGlobe, which serve a variety of markets beyond agriculture have promised to fill the gaps made from the public-sponsored platforms. However, the cost and complexity of using these data have been limiting factors at the producer level for all but the large farm operations who can justify data costs. As a result much of the use of remote sensing data has come in the past from aerial platforms and now from drones carrying small sensors focused on frequent observations over the growing season. Although at a limited coverage area, the cost of entry can be considered high for smaller farms operations in the US and, especially in the developing world creating a logistics problem of sensor and data exchange.
Poor Communication Infrastructure
In addition to the difficulty arising from a variety of remote sensing data from multiple sensors, the lack of a comprehensive backbone for high-bandwidth transmission of data to remote farm areas has limited the ability of the exchange of data between the farm and value-added services. In the US broadband adoption promises to solve this problem with bandwidth at the megabyte level, but small farm operations in low-income countries will have to rely on alternative architectures that utilize local sensor networks. Tyler and Griffin (2016) argues that “The realization of ‘Big Data's’ value will not happen until [the data transfer bandwidth] barrier is overcome.” On the surface, PA looks to not be a big generator of data for upload. Tyler and Griffin (2016) suggests otherwise for just corn alone, where for each plant generating 0.5 k bytes, the 88.9 million acres in 2015 would generate 1.3 petabytes of data, not including the notorious big data generator from aerial drones. Upload bandwidth will clearly be needed.
Siloed Data Management
Given the variety of data and limitations of bandwidth in many farming communities, it's not surprising that data management has also been an issue. First, the public remote sensing platforms, whose data generation capabilities have led to data archives at the exabyte (i.e., 1018 bytes) and above levels, have resulted in driving the public sector to invest into large data systems in order to serve a wide scientific community. These systems, while good at disseminating data, still require extensive and complex knowledge of a variety of satellites and sensors, file formats, meta data standards, physics, etc. ( Blumenfeld, 2019 ). In short, it still does require considerable expertise to gain any significant agricultural benefits from these systems.
Second, commercial satellite, aerial, and drone-based data systems, while somewhat easier to use, are still fragmented due to competing interests. In the end, the variety of data formats, velocity of data coming off of a variety of platforms, and volume of data generated have led to a fragmented and siloed data management infrastructure for agriculture. In other words, this is a Big Data problem, which is formally defined as a combination of a variety of data, the velocity of data and/or the volume of data.
Given the inherently spatial nature of agriculture and remotely sensed data, GIS offered the opportunity to minimize data siloes by providing spatial context (i.e., maps) around data. This has led to a proliferation of GIS systems that span topic areas well beyond remotely sensed data, yet has led to additional silos of geospatial information.
Immature Applications of Analytics and AI
While data silos are not inherently a problem per se , techniques including those from analytics, AI and Machine Learning, require that the data be readily accessible, coherent, and consistent before these algorithms can provide any value. Certainly, first-generation solutions like the world wide web and data warehouses have gone a long way in connecting these data siloes, but they fell short in addressing the computational capacity necessary for analytic techniques. These challenges made it difficult to apply analytic techniques comprehensively such as yield forecasting and all the other advanced techniques tried in the scientific literature.
The problem is further exacerbated when considering SPAE and modeling. In PA, applications focus on connection of inputs to needs in order to determine the action based on a spatial area. That is, while there is some modeling going on, it's relatively simple in that a precision map shows exactly where to apply the fertilizer, water, etc. SA, which places emphasis on complex interactions between biological systems, tends to have more complex models that are crafted carefully by the scientific field. Consider the Denitrification and Decomposition (DNDC) model used in the simulation of carbon and nitrogen biogeochemistry through complex interactions of soil, crop growth/decomposition, etc. to predict, among other things, nitrate leaching and C/N greenhouse gas emissions ( Salas, 2010 ; University of New Hampshire, 2012 ). Whereas initial PA efforts may not be as focused on assessing greenhouse emissions from agriculture, this more complicated model or analytic technique is an example of one that could contribute to achieving the objectives of SPAE and, as such, requires a strong source of “regional databases…for mapping and potentially monitoring management practices…for compliance, verification, or tracking sustainability” ( Salas, 2010 ).
Another recent example is the COMET-Farm system web-based tool developed by Paustian et al. (2018) to do full greenhouse gas assessments for CO 2 , CH 4 , and N 2 O from all major on-farm emission sources as well as assessments of soil carbon sequestration. This web-based tool can assess GHG emissions from perennial crops, pasture, range and agroforestry systems, as well as emissions from livestock and on-farm energy use ( Paustian et al., 2018 ). This tool can be used to assess PC practices. The user could upload a custom soil map that can specify sub-field map units, allowing the user to define spatially explicit management zones ( Paustian et al., 2018 ). An application could be added in the future to assess the effects of PA and PC on GHG emissions ( Paustian et al., 2018 ). Saleh and Osei (2018) reported that the Comprehensive Economic Environmental Optimization Tool (CEEOT) and the more user-friendly Nutrient Tracking Tool (NTT) can be used at a watershed level to assess the optimal conservation practices, using a PC approach to maximize the benefit from each dollar of conservation practice investment. They reported that by using spatial distributions of field attributes such as soil type, topography, and soil chemical and physical properties, PC can be applied to reduce the uncertainty of where to apply a given practice to increase conservation effectiveness and thus sustainability ( Saleh and Osei, 2018 ).
Regional databases are equally as critical for building accurate machine-learning algorithms. In the geospatial world, early applications of AI focused on classification of imagery such as Landsat ( Campbell et al., 1989 ), which led to applications mostly around macroeconomic crop forecasting, as is done by the United States Department of Agriculture's (USDA) National Agricultural Statistics Service. Figure 2 illustrates the problem through the classifications of cropland (yellow), forest (green), and roadways (gray) in the left picture, where even a casual inspection shows misclassification of the entire farm in the polygon. When taken at a national or global level, these classification error rates may well be within statistical tolerances for macroeconomic problems, but clearly fertilizing the road or farm buildings would not be acceptable in the left picture. The picture to the right on higher resolution data (NAIP) from a recent use of machine learning in the cloud shows drastically improved classification ( Tayyebi, 2019 ) due to not only higher spatial resolution data but also significant computational capacity in today's cloud environment.
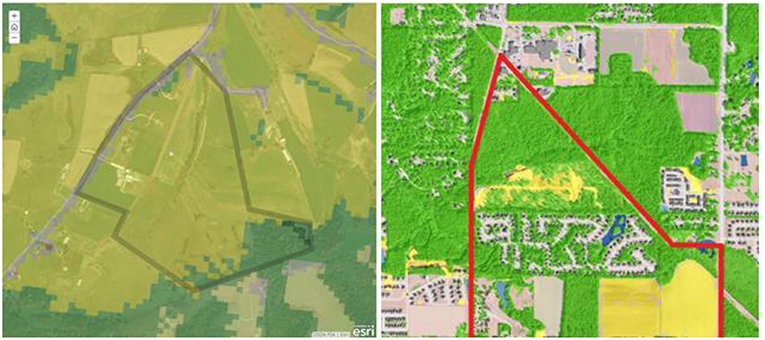
Figure 2 . Coarse classification (left) vs. recent AI classification methods (right) ( Tayyebi, 2019 ).
In either case, Campbell et al. (1994) and Short et al. (1995) recognized early that regional databases needed to be developed as a mechanism for providing training data to machine-learning algorithms, which capture local knowledge from different individuals or organizations (e.g., farmers). In other words, these classification techniques rely heavily on supervised learning where training datasets consisting of known classification labels associated with spectral properties (i.e., spectral signature) are presented to the algorithms, which generalize or learn.
In the 1990s, NASA recognized the need to develop a network of distributed, end-to-end satellite processing centers, called Regional Application Centers, in order to leverage local knowledge in the building of training datasets for localized machine-learning implementations. Implemented around the world in countries with no interconnectivity at the time (i.e., the internet), NASA deployed low-cost, high-performance technology to acquire data directly from the satellites with direct broadcast capability and perform all the necessary processing routines including machine learning, in order to produce spatial information products for not only NASA but also the local and international governments ( Campbell et al., 1994 ; Davis et al., 1994 ; Short and Dickens, 1995 ). Because the mission areas ranged from hurricane forecasting to early applications of PA, the network of regional data centers demonstrated a coexistence between science goals and operation mission effectiveness that resulted in, for example, an “early warning [that] allowed the movement of over 200,000 people [in Bangladesh] to higher elevation thereby avoiding certain drowning due to flooding” ( Campbell et al., 1994 ).
The Paradigm Shift: Digital Agriculture
Research and industry could solve many of these problems given the inherent nature of PA as a simple business solution of minimizing input costs through big data management in order to maximize yield and profits in a commodity-based industry. In a recent Bloomberg article ( Noel, 2019 ) argues that companies like Bayer can acquire data from the farm, process it with analytics, and sell it back to the producers. With advances in the cloud where computational power and storage are relatively inexpensive, companies will probably move into a new era of selling information products by coalescing many of the aforementioned data sources, thereby reducing siloes and the knowledge complexity of operating the variety of data generators.
However, SPAE is different due to its focus on more environmentally friendly techniques and reliance on natural farming practices that require a level of complexity beyond simple input/outcome optimizations. Not only are the techniques less scientifically understood, but the potential improvements in yield may not generate the economic benefits required to justify the increased complexity and costs in the short run, such as the aforementioned analytic complexity for SPAE alone. With that said, recent advances in PC to increase SPAE has shown that there is potential for some quick profits when conservation practices use these new technologies. For example, Thompson and Sudduth (2018) reported that using PA tools offers an advantage in both designing and utilizing terracing and contour farming approaches to conservation management by reducing terrace layout time by 50%, contributing to savings in time and money. Thompson and Sudduth (2018) also reported that using PA will contribute to economic sustainability of these systems by using field machines that utilize GPS guidance and automatic row or section control.
New opportunities may also be possible if ecosystem markets are developed where nitrogen, carbon, or even reduction of GHG can be traded in market systems, providing additional income to farmers due to implementation of conservation practices ( Ribaudo et al., 2005 , 2011a , b ; Delgado et al., 2008 , 2010 ; Paustian et al., 2018 ; Saleh and Osei, 2018 ). Paustian et al. (2018) reported that GHG mitigation, carbon storage, and water filtration, for example, could potentially be appreciated and monetized, but it will then bring greater complexity to the decision-making and management choices of farmers and ranchers; it will also require tools and technologies that have not previously been available to agricultural producers. Delgado et al. (2008 , 2010 ) reported that a GIS nitrogen trading tool could potentially assess the spatial effects of implementation of conservation practices on reductions in nitrate leaching and direct and indirect GHG emissions that could be traded in air and water quality markets. Saleh and Osei (2018) reported that we could use spatial tools to assess the effects of conservation practices and how PC could be used to generate credits for water quality markets and to specify, for example, if the credits will be for sediment load reduction or nitrogen or phosphorus reduction. Saleh and Osei (2018) reported that by using PC there will be a reduction in the “margin of safety” credit adjustments necessary for water quality trades, improving the water quality trading options for farmers. As suggested in Campbell et al. (1994) around Distributed AI techniques for automated cooperative systems, we propose that by using AI and the new SPAE approach and technology there will be greater opportunities to make SPAE generate income in these trading systems for farmers and ranchers, and increase field, watershed, national, and global conservation.
The rest of this paper argues that big data analytics is at the core of combining precision and sustainability into an earlier notion of Sustainable Agriculture ( Berry et al., 2003 , 2005 ; Bongiovanni and Lowenberg-DeBoer, 2004 ; Delgado and Berry, 2008 ). Berry et al. (2003) reported that precision conservation will be needed to maintain the productivity of intensive agricultural systems and global sustainability. Berry et al. (2003) also reported that precision conservation has the potential to integrate conservation practices at a site-specific field level with off-site conservation practices, which would contribute to watershed sustainability. To support this argument, we will focus on both farm management and a geoinformatics cloud framework as a step toward global agricultural sustainability.
Part of the PA concept to increase the efficiency of fertilizers has been the use of a 4 Rs approach to reduce nutrient losses from farming systems (the right product, at the right rate, at the right time, and at the right place ( Roberts, 2007 ). However, Delgado (2016) reported that the 4 Rs are not enough and gave examples where significant losses of nutrients and soil could occur without precision conservation ( Figure 3 ). The concept of agricultural sustainability using a precision conservation approach in agriculture presented by Berry et al. (2003) will contribute to reduced off-site impacts across a watershed by using these new technologies to improve the design of conservation practices and increase the efficiencies of conservation practices such as field buffers, sediment traps, denitrification traps, and riparian buffers to minimize the losses of nutrients from the field and across a watershed. Cox (2005) reported that the Berry et al. (2003) PC concept could also be described using a 4 Rs approach, by applying the right conservation practice, at the right place, at the right time, and at the right scale (the 4 Rs of conservation). Delgado (2016) combined these 4 Rs of precision farming with the 4 Rs of precision conservation to create the 7 Rs for nutrient management and conservation, which are applying the right product (1), the right rate (2) and the right method of fertilizer application (3), the right conservation practice (4) placed at the right place (5) and right scale (6), with the right time of application of fertilizer and establishment of the conservation practice (7). We propose that we could use new Big Data analytics to combine precision agriculture and precision conservation ( Berry et al., 2003 , 2005 ; Bongiovanni and Lowenberg-DeBoer, 2004 ; Delgado and Berry, 2008 ) to achieve SPAE. This is similar to the 7 Rs approach described by Delgado (2016) and Sassenrath and Delgado (2018) .
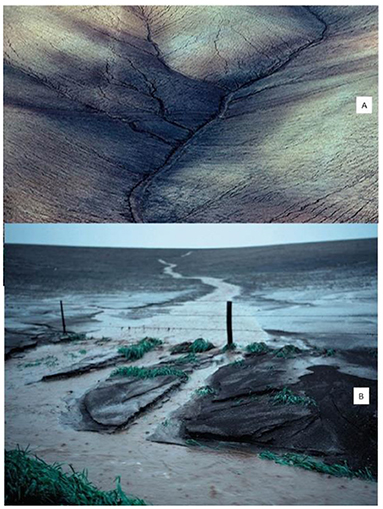
Figure 3 . Locations (A,B) where the 4 Rs alone would not reduce the off-site transport of nutrients and a 7 Rs approach would contribute to reducing off-site transport of nutrients (Images from NRCS showing development of ephemeral gullies).
Modern Farm Management
With the emergence of commercial viability of PA over the past few decades, today's developed world farmer is moving from a grower and distributor of produce to a modern day data scientist who must utilize analytic techniques to both collect the right data at the right time, but also apply advanced information products to increase yield. Acting in the role of an early adopter of technology ( Moore, 1991 ), today's farmer will have to quickly learn how these new technologies can be used to help make decisions about how to increase profits by increasing yields or implementing precision management and conservation practices that could produce sustainability benefits that could potentially be traded in ecosystem service markets. The use of this new technology for SPAE will be dominated in the future by analytic techniques and AI to help provide solutions to complex problems and decisions.
Today's “early majority” farmer is increasingly aware of the role of cost externalities as they relate to input costs and will act as the bridge from the early adopter technology community. For example, whether it is organic farms that must deal with cross-species contamination from neighboring farms or the increased externalities from management practices that contribute to emissions of GHG or losses of agrochemicals that could impact water quality, or weather impacts on erosion and loss of productivity, farming must increasingly deal with long-term factors that may not show up immediately in increased yield profits but that will contribute to reduced yields if they remain unchecked. Using precision conservation to increase the sustainability of agricultural systems will contribute to adaptation to a changing climate and maintaining long-term productivity. In other words, sustainability in agriculture is increasingly becoming a necessary component of today's agricultural practices. Organizations like USDA's Natural Resources Conservation Service (NRCS) have long known that conservation programs are methods for removing the cost burden of externalities, but with decreased public sector budgets, this is becoming more difficult as external costs increase.
Managing nutrients at a farm level is very important since losses of reactive nitrogen at this level significantly impact the environment via emissions of nitrous oxide, ammonia emissions, nitrate leaching losses, and off-site transport of surface losses of nitrogen ( Smith et al., 1997 ; U.S. Environmental Protection Agency, 2010 ). Nitrogen losses significantly impact terrestrial resources, water bodies, and the atmosphere ( Hutchinson et al., 1982 ; Legg and Meisinger, 1982 ; Cowling et al., 2002 ; Rabalais et al., 2002a , b ; Galloway et al., 2008 ; Dubrovsky et al., 2010 ). It has been documented that these losses contribute to significant impacts such as impacts on species composition and the functioning of terrestrial, freshwater, and marine ecosystems, among others ( Matson et al., 1997 ; Vitousek et al., 1997 ). Reactive nitrogen could also negatively impact human health ( Follett et al., 2010 ). In addition to significant environmental impacts, these losses can also have negative economic impacts. Ribaudo et al. (2011a) reported that the cost of removing agriculture's contribution to nitrate loadings in drinking water in the USA is about $1.7 billion per year.
Fortunately, organizations like USDA's NRCS and private consultants have been increasing the use of these new technologies that contribute to implementation of conservation practices on the ground to achieve precision conservation. Precision conservation is increasingly being embraced by agencies such as NRCS and the private sector in a new revolutionary approach that is increasing conservation effectiveness ( USDA-NRCS, 2017 ). Some of the USDA ARS' voluntary conservation programs such as the Environmental Quality Incentives Program (EQIP) can be used to implement precision conservation (USDA-NRCS, USDA-NRCS). There are several examples of private sector and non-profit organization implementation of precision conservation to increase sustainability ( Buman, 2016a , b ; Hammes, 2016 ; Heartland Science Technology Group, 2017 ; Chesapeake Conservancy, 2018 ; Illinois Sustainable Ag Partnership, 2018 ).
There is a revolution going on in the agricultural landscape, making it possible to move agricultural science from on-site research facilities directly to the farm. Farmers are no longer passive recipients of information but are rather actively involved in the science and development of new crop production systems that can either be yield neutral or possibly improve yield. Over the long run, this paradigm shift could reduce the need for public subsidies for external costs, as they would be an automatic byproduct of agricultural best practices.
As suggested in Wolfert et al. (2017) ( Figure 4 ), the key to the modern farm is the application of big data to the development of a smart farm and smart soil and water conservation ( Sassenrath and Delgado, 2018 ). The smart farm and smart soil and water conservation conceptual frameworks focus on the cyber-physical management cycle built around a cloud-based infrastructure that manages all farm operations. Where bandwidth is plenty (i.e., due to rural broadband), then the farmer operates as a data collector transmitting to the cloud for spatially-based analytic techniques that tell the farmer what to do and when based on looking at the macro variables across the farm landscape (e.g., weather input prediction).
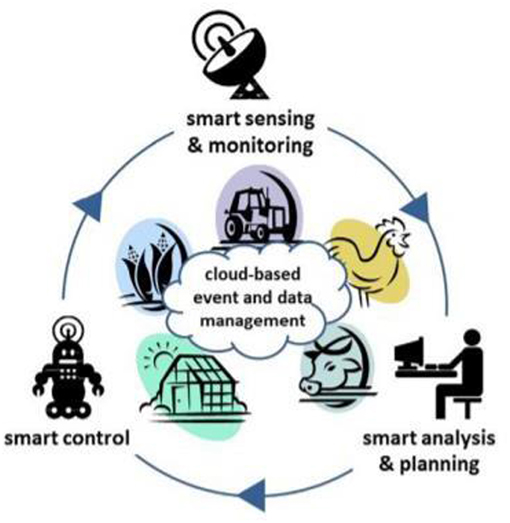
Figure 4 . The smart farm conceptual framework ( Wolfert et al., 2017 ). © The Authors. Published by Elsevier Ltd. This is an open access article under the CC BY-NC-ND license ( http://creativecommons.org/licenses/by-nc-nd/4.0/ ).
For agriculture in the developed world, Unmanned Aerial Vehicles (UAV) and Unmanned Ground Vehicles (UGV) armed with hyper-spectral and other sensor types generate significant amounts of data that can be processed remotely enabling a variety of advanced analytic techniques that can drive the application of inputs automatically. Computer visions techniques are increasingly being used on applications like weed vs. crop detection ( Lin et al., 2017 ), planting, irrigation, pruning, harvesting, and plant disease detection/identification ( Ampatzidis et al., 2017 ), leading to the potential for further automation and minimizing of labor input costs.
Where bandwidth is limited, especially in the developing world, computation must be pushed to the edge where information products are produced in situ and only small data volume information products are exchanged between the cloud and farmer if at all. Referred to as edge computing, this “distributed computing paradigm….brings computer data storage closer to the location where it is needed” (Edge Wiki) 2 and is possible to the drastic drops in price/performance for hardware. In the context of agriculture, this means that machine learning algorithms that are developed in the cloud can be pushed to the edge for farm operations.
A powerful example of this model can be found in Microsoft's FarmBeats program ( Vasisht et al., 2017 ). Rather than wait for governments or private industry to invest in connectivity programs like rural broadband, FarmBeats “piggy backs” off of T.V. whitespaces 3 , which are frequencies allocated to broadcasting service but not locally used, especially in rural areas. FarmBeats deploys a field sensor array to collect variables (e.g., soil moisture) where the machine learning is used to impute or predict data points from sensor nodes and brief fly overs from UAVs. By building on inexpensive and reliable hardware, FarmBeats addresses the “late majority's” market need for simplicity and low risk, thereby facilitating technology adoption ( Moore, 1991 ). As a result, FarmBeats has already been deployed internationally in countries like India, New Zealand, and Kenya, resulting in a reported 30% reduction in water consumption ( Sims, 2019 ).
Based on trends in farm operations and management, farms are becoming the new Big Data generator that complements much of the EO data that has been gathered to date. With these advances architecturally in farm management, the modern farm can resemble the notion of “Digital Twins”, which is the confluence of IoT, AI, and big data. [Digital Twin Wiki] 4 defines a digital twin as “a digital replica of a living or non-living physical entity” that is used “to create living digital simulation models that update and change as their physical counterparts change.” In terms of agriculture and farm management, digital twins means that “farm operations would no longer require physical proximity, which allows for remote monitoring, control and coordination of farm operations” ( Verdouw and Kruize, 2015 ). From a SPAE perspective, simulation models like the aforementioned DNDC, COMET-Farm, CEEOT, and/or the NTT models are among some of the available tools that could be used to form the basis of ensuring the least impact on environment without driving up costs.
A Geoinformatics Sustainable Agriculture Framework
Regardless of the approaches, clearly today's farms are quickly becoming data generators that when stitched together spatially provide a higher resolution view into the agricultural industry, which ultimately will provide a more precise view of not only global food security but overall environmental sustainability. Governments currently have to rely heavily on EO data to estimate crop yield as it relates to food security and market predictions, which is an indirect indicator of sustainability more from an economic than environmental perspective. This has certainly been the goal of USDA's Global Agricultural & Disaster Assessment System (GADAS), which uses satellite imagery “to assist in…agricultural estimates of global crop conditions” ( Frantz, n.d. ).
GADAS represents a first-generation system that potentially forms the foundation for a broader public/private partnership of connecting farm data generators to an interconnected framework for sustainability. Or namely, it brings together a variety of spatial data via a GIS to illustrate impacts of weather, water, crop conditions, land use, etc. to give a global assessment of agriculture ( Figure 5 ). In fact, the US Federal government is starting to recognize the need for governance of geospatial data from a comprehensive point-of-view. Through the Geospatial Data Act (GDA), the intent of the act is to “coordinate and work in partnership with other Federal agencies, agencies of State, tribal, and local governments, institutions of higher education, and the private sector to efficiently and cost-effectively collect, integrate, maintain, disseminate, and preserve geospatial data, building upon existing non-Federal geospatial data to the extent possible” ( URISA, 2018 ). In other words, the GDA provides a governance framework for bringing together billions of dollars of investment in geospatial data from a variety of environmental and military mission areas.
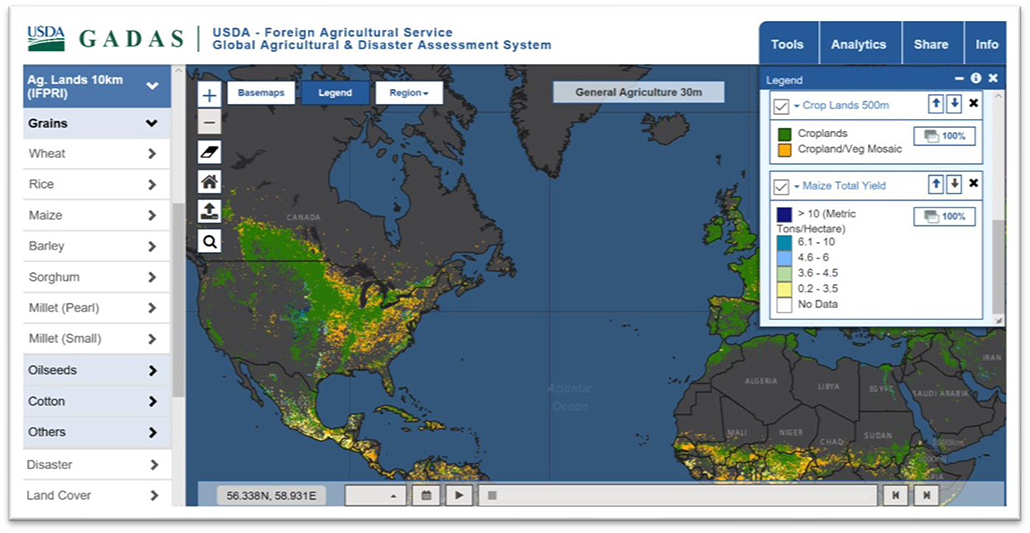
Figure 5 . The Global Agricultural and Disaster Assessment System (GADAS) is a GIS system that provides data about global crop conditions based on satellite imagery and remotely sensed data [USDA-FAS].
Jack Dangermond from Esri established the vision for a geospatial infrastructure so that “users can easily and inexpensively access an immense wealth of geographic information on almost any subject…[leveraging] cloud computing resources to perform analysis and mapping” ( Dangermond, 2018 ). Technologically, this means providing a platform for connecting existing GIS systems together into a new architectural pattern referred to as WebGIS. The WebGIS pattern supports multiple implementation patterns from the on Farm, edge-oriented architecture presented in the previous section to a “system of systems” spread across the private and public sector.
From an agricultural perspective, WebGIS provides a framework for reducing past siloes not only across the public sector agencies, but also between the public sector and agricultural industry. USDA's Agricultural Research Service (ARS) has taken a lead in breaking down these silos by leveraging WebGIS. Although in its early stages, the Agricultural Collaborative Research Outcome System (AgCROS) illustrates the vision of collaboration by providing a single platform for the dissemination of new agricultural scientific discoveries and techniques ( Delgado et al., 2018b ). AgCROS was built based on individual ARS national research projects. These projects studied areas such as greenhouse gas emissions, soil health, genomics, cover crops, renewable energy, antibiotic resistance, nutrient use and nutrition ( Del Grosso et al., 2013 ; Delgado et al., 2016 ; Delgado et al., 2018b ).
Sustaining the Earth's Watersheds, Agricultural Research Data System (STEWARDS) started the ARS multilocation national natural resource projects. STEWARDS used what is now called WebGIS, but each location had its own measurement vocabulary, so location cross comparison was not possible ( Steiner et al., 2009 ). STEWARDS did introduce a measurement methods catalog which is a very important component in any of these systems. The Greenhouse gas Reduction through Agricultural Carbon Enhancement network (GRACEnet) was the first project to show true collaboration among scientists ( Del Grosso et al., 2013 ). GRACEnet, also used a WebGIS. GRACEnet showed that through collaboration among the scientists, a way to build up a common vocabulary of measurements for greenhouse gas emissions. This has been key in building systems around each of these individual projects since for ARS. GIS provided a way to relate all this data based on location. The addition of metadata for public discovery and measurement methods now allow the individual location data to be combined to look at trends across ARS research locations. The next steps for AgCROS will be adding imagery, real time sensors, and electronic field collection to allow more data to be added with the goal of allowing the machine learning techniques discussed above to be realized. More importantly, it promotes the integration of agricultural knowledge using the WebGIS pattern in order to promote collaboration across government, industry and academia. In other words, it is the mechanism for speeding up the adoption of new crop production techniques to the smart farm, which can in turn deliver higher quality data back to the scientific community for enabling sound science. In effect, these aforementioned systems along with a smart farm network suggest that WebGIS could, in fact, become the Digital Twin for the Globe. In this way, such a system would facilitate the transparency of environmental costs and reduce cost externalities based on real-data from monitoring systems down to the farm/producer, thereby enabling more data-driven policy making.
Precision Agriculture emerged out of the 1980s because of the development of several key technologies as a way to improve margin through cost management of inputs while improving yield. Development of precision-conservation practices started in the early 2000s. New technologies like GPS, satellite imagery, and new methods of genetic modification in the green revolution have represented a disruption in agriculture not seen since the introduction of the first successful commercial tractor in the early 1900s and the green revolution that occurred between 1950 and the late 1960s. With the increasing impact of climate change, this paper has argued that the next revolution in precision agriculture will be driven by SPAE which could leverage past technologies combined with Big Data analysis. Among other positive impacts, SPAE will contribute to increased yields and profits, increased adaptation to a changing climate, increased sustainability of agricultural systems, and increased sustainability outside of the field and across watersheds, reducing nutrient transport across watersheds and contributing to global sustainability.
While the traditional definition of sustainable agriculture focused on incorporating new practices that deal with ecosystem services, this new, technology-focused sustainable agriculture transitions from a site-specific management focus to the notion of global sustainability. To accomplish this transition, we introduced the WebGIS framework as an organizing principle that connects local, site-specific data generators called smart farms to a regional and global view of agriculture that can support both the agriculture industry and policy makers in government.
Automation and the use of AI, IoT, drones, robots, and Big Data serve as a basis for “Digital Twins,” which could allow for simulations of new ideas that can be tested virtually to determine environmental impact before implementation in the real world. In other words, constructing new practices in the virtual world will reduce the time to deploy new practices that lead to better environmental outcomes. If we are to feed 10 billion people by 2100 while preserving our environment, the next green revolution must incorporate the virtual world.
Author Contributions
All authors listed have made a substantial, direct and intellectual contribution to the work, and approved it for publication.
Trade and manufacturer's names are necessary to report factually on available data; however, the USDA neither guarantees nor warrants the standard of the product, and the use of the name by USDA implies no approval of the product to the exclusion of others that may also be suitable. USDA is an equal opportunity provider and employer.
Conflict of Interest Statement
The authors declare that the research was conducted in the absence of any commercial or financial relationships that could be construed as a potential conflict of interest.
Acknowledgments
The authors would like to extend a special thanks to Dr. Charlie Walthall for inspiring this work and improving our knowledge of airplanes. We would also like to thank Jack Dangermond and Nicholas Short, Sr. for long ago inspiring us to go into this field.
1. ^ Applying GIS and remote sensing to landscape genetics and genome size research. Available online at: https://pdfs.semanticscholar.org/3054/4c8c85b5bfc7e3f07725329376120473178a.pdf
2. ^ Edge computing Wiki. https://en.wikipedia.org/wiki/Edge_computing
3. ^ Adoption of computer based information systems: The case of dairy farmers in Canterbury, NZ, and Florida, Uruguay. Available online at: https://mentor.ieee.org/802.22/dcn/19/22-19-0013-00-0000-tutorial-on-whitespaces.pdf
4. ^ Digital Twin Wiki. Available online at: https://en.wikipedia.org/wiki/Digital_twin
Ampatzidis, Y., De Bellis, L., and Luvisi, A. (2017). iPathology: robotic applications and management of plants and plant diseases. Sustainability 9:1010. doi: 10.3390/su9061010
CrossRef Full Text | Google Scholar
Antle, J., Jones, J., and Rosenzweig, C. (2017). Next Generation agricultural system data, models and knowledge products: introduction. Agric. Sys . 155, 186–190. doi: 10.1016/j.agsy.2016.09.003
PubMed Abstract | CrossRef Full Text | Google Scholar
Beal, G., and Bohlen, J. (1957). The Diffusion Process . Special Report No. 18. Iowa Agricultural and Home Economics Station Publications, Iowa State University.
Google Scholar
Berry, J. K., Delgado, J. A., Khosla, R., and Pierce, F. J. (2003). Precision conservation for environmental sustainability. J. Soil Water Conserv . 58, 332–339.
Berry, J. K., Delgado, J. A., Pierce, F. J., and Khosla, R. (2005). Applying spatial analysis for precision conservation across the landscape. J. Soil Water Conserv . 60, 363–370.
Blumenfeld, J. (2019). Meeting Data User Needs: A Look Behind the Curtain . Available online at: https://earthdata.nasa.gov/meeting-data-user-needs
Bongiovanni, R., and Lowenberg-DeBoer, J. (2004). Precision agriculture and sustainability. Prec. Agric . 5, 359–387. doi: 10.1023/B:PRAG.0000040806.39604.aa
Bouis, H., and Saltzman, A. (2017). Improving nutrition through biofortification: a review of evidence from HarvestPlus, 2003 through 2016. Global Food Sec . 12, 49–58. doi: 10.1016/j.gfs.2017.01.009
Buman, T. (2016a). Testimony of Tom Buman, CEO of Agren. For the House Agriculture Sub-Committee on Conservation Forestry. HearingTopic: Solutions Through Voluntary and/or Locally Led Conservation Efforts . Washington, DC: House Committee on Agriculture. Available online at: https://agriculture.house.gov/uploadedfiles/buman_testimony.pdf (accessed August 31, 2018).
Buman, T. (2016b). Voluntary Conservation Solutions . Carroll, IA: Agren Tools. Available online at: https://precisionconservation.com/voluntary-conservation-solutions/ (accessed August 30, 2018).
Campbell, W., Hill, S., and Cromp, R. (1989). Automatic labeling and characterization of objects using artificial neural networks. Telemat. Inform. 6, 259–271. doi: 10.1016/S0736-5853(89)80021-8
Campbell, W., Short, N., Coronado, P., and Cromp, R. (1994). “Distributed earth science validation centers for mission to planet earth,” in Methodologies for Intelligent Systems. ISMIS 1994. Lecture Notes in Computer Science (Lecture Notes in Artificial Intelligence), Vol. 869 , eds Z. W. Raś and M. Zemankova (Berlin; Heidelberg: Springer), 1–12.
Chesapeake Conservancy (2018). Precision Conservation . Annapolis, MD: Chesapeake Conservancy. Available online at: http://chesapeakeconservancy.org/conservation-innovation-center/precision-conservation/ (accessed August 30, 2018).
Cowling, E., Galloway, J., Furiness, C., Erisman, J. W., et al. (2002). “Optimizing nitrogen management and energy production and environmental protection,” in Presented at the Second International Nitrogen Conference (Potomac, MD: Bolger Center). Available at www.initrogen.org/fileadmin/user_upload/Second_N_Conf_Report.~pdf
Cox, C. (2005). Precision conservation professional. J. Soil Water Conserv . 60:134A.
Dangermond, J. (2018). “The Emergence of a Geospatial Cloud and the Continuing Evolution of Cloud Computing”, ArcNews , Spring 2019, Vol. 41, No. 2.
Davis, D., Bennett, T., and Short, N. Jr. (1994). “A low-cost transportable ground station for capture and processing of direct broadcast EOS satellite data,” in Proceedings from the 3rd International Symposium on Space Mission Operations and Ground Data Systems, Space Ops '94, Part 1 (Greenbelt, MD), 187–195.
Del Grosso, S. J., White, J. W., Wilson, G., Vandenberg, B., Karlen, D. L., Follett, R. F., et al. (2013). Introducing the GRACEnet/ REAP data contribution, discovery, and retrieval system. J. Env. Qual . 42, 1274–1280. doi: 10.2134/jeq2013.03.0097
Delgado, J. A. (2016). “4 Rs are not enough. We need 7 Rs for nutrient management and conservation to increase nutrient use efficiency and reduce off-site transport of nutrients,” in Soil Specific Farming: Precision Agriculture. Advances in Soil Science Series , eds R. Lal and B. A. Stewart (Boca Raton, FL: CRC Press, 89–126.
Delgado, J. A., and Berry, J. K. (2008). Advances in precision conservation. Adv. Agron . 98, 1–44. doi: 10.1016/S0065-2113(08)00201-0
Delgado, J. A., Groffman, P. M., Nearing, M. A., Goddard, T., Reicosky, D., Lal, R., et al. (2011). Conservation practices to mitigate and adapt to climate change. J. Soil Water Conserv . 66, 118A−129A. doi: 10.2489/jswc.66.4.118A
Delgado, J. A., Gross, C. M., Lal, H., Cover, H., Gagliardi, P., McKinney, S. P., et al. (2010). A new GIS nitrogen trading tool concept for conservation and reduction of reactive nitrogen losses to the environment. Adv. Agron . 105, 117–171. doi: 10.1016/S0065-2113(10)05004-2
Delgado, J. A., Sassenrath, G., and Mueller, T. (ed.) (2018a). Precision Conservation: Geospatial Techniques for Agricultural and Natural Resources Conservation. Agronomy Monograph 59. Madison, WI: ASA, CSSA, and SSSA.
Delgado, J. A., Shaffer, M. J., Lal, H., McKinney, S. P., Gross, C. M., and Cover, H. (2008). Assessment of nitrogen losses to the environment with a nitrogen trading tool (NTT). Comput. Electron. Agric . 63, 193–206. doi: 10.1016/j.compag.2008.02.009
Delgado, J. A., Vandenberg, B. C., Kaplan, N. E., Neer, D. L., Wilson, G. J. D., et al. (2018b). Agricultural collaborative research outcomes system: AgCROS - An emerging network of networks for national food and environmental security and human health. J. Soil Water Conserv . 73, 158A−164A. doi: 10.2489/jswc.73.6.158A
CrossRef Full Text
Delgado, J. A., Weyers, S., Dell, C., Harmel, D., Kleinman, P., Sistani, K., et al. (2016). USDA agricultural research service creates Nutrient Uptake and Outcome Network (NUOnet). J. Soil Water Conserv . 71, 147A−148A. doi: 10.2489/jswc.71.6.147A
Dolan, M., Holden, C., Beard, M., and Bult, C. J. (2006). Genomes as geography: using GIS technology to build interactive genome feature maps. BMC Bioinform . 7:416. doi: 10.1186/1471-2105-7-416
Dubrovsky, N. M., Burow, K. R., Clark, G. M., Gronberg, J. A. M., Hamilton, P. A., Hitt, K. J., et al. (2010). The Quality of Our Nation's Waters - Nutrients in the Nation's Streams and Groundwater, 1992-2004 . Circular-1350. U.S. Geological Survey, Sacramento, CA.
Follett, J. R., Follett, R. F., and Herz, W. C. (2010). “Environmental and human impacts of reactive nitrogen,” in Advances in Nitrogen Management for Water Quality , eds J. A. Delgado and R. F. Follett (Ankeny, IA: Soil and Water Conservation Society) 1–37.
Frantz, R. (n.d.). About Global Agriculture and Disaster Assessment System. Available online at: https://geo.fas.usda.gov/GADAS/pageDirectories/about/about.html
Fulton, J., and Darr, M. (2018). “GPS, GIS, guidance, and variable rate technologies for conservation management,” in Precision Conservation: Geospatial Techniques for Agricultural and Natural Resources Conservation. Agronomy Monograph 59 , eds J. Delgado, G. Sassenrath, and T. Mueller (Madison, WI: ASA, CSSA, and SSSA, 65–82.
Galloway, J. N., Townsend, A. R., Erisman, J. W., Bekunda, M., Cai, Z., Freney, J. R., et al. (2008). Transformation of the nitrogen cycle: recent trends, questions, and potential solutions. Science 320, 889–892. doi: 10.1126/science.1136674
Gebbers, R., and Adamchuk, V. (2010). Precision agriculture and food security. Science 327, 828–831. doi: 10.1126/science.1183899
Goolsby, D. A., Battaglin, W. A., Aulenbach, B. T., and Hooper, R. P. (2001). Nitrogen input to the Gulf of Mexico. J. Environ. Qual . 30, 329–336. doi: 10.2134/jeq2001.302329x
Hammes, A. (2016). Precision Conservation Management Program Receives National Grant. Ames, IA: AgSolver . Available online at: https://medium.com/@amber_hammes/precision-conservation-management-program-receives-national-grant-97404c374ca2
Heartland Science Technology Group (2017). Precision Conservation Management. Champaign, IL: Heartland Science and Technology Group . Available online at: https://farmerportal.precisionconservation.org/authentication/signup
Hutchinson, G. L., Mosier, A. R., and Andre, C. E. (1982). Ammonia and amine emissions from a large cattle feedlot. J. Env. Qual. 11, 288–293. doi: 10.2134/jeq1982.00472425001100020028x
Illinois Sustainable Ag Partnership (2018). Precision Conservation Management. East Peoria, IL . Available online at: http://ilsustainableag.org/programs/precision-conservation-management/ (accessed August 30, 2018).
Jenrich, M. (2011). Potential of precision conservation agriculture as a means of increasing productivity and incomes for smallholder farmers. J. Soil Water Conserv . 66, 171–174. doi: 10.2489/jswc.66.6.171A
Kuehne, G., Llewellyn, R., Pannell, D., Wilkinson, R., Dolling, P., Ouzman, J., et al. (2017). Predicting farmer uptake of new agricultural practices: a tool for research extension and policy. Agricult. Sys . 156, 115–125. doi: 10.1016/j.agsy.2017.06.007
Legg, J. O., and Meisinger, J. J. (1982). “Soil nitrogen budgets,” in Nitrogen in Agricultural Soils, Agronomic Monograph 22 , ed F. J. Stevenson (Madison, WI: American Society of Agronomy, 503–557.
Lin, F., Zhang, D., Huang, Y., Wang, X., and Chen, X. (2017). Detection of corn and weed species by the combination of spectral, shape, and textural features. Sustainability 9:1335; doi: 10.3390/su9081335
Luccio, M. (2014). Satellite Imagery for Precision Agriculture . Available online at: https://www.xyht.com/enviroag/satellite-imagery-precision-agriculture/
Matson, P. A., Parton, W. J., Power, and Swift, M. J. (1997). Agricultural intensification and ecosystem properties. Science 277, 504–509. doi: 10.1126/science.277.5325.504
McConnell, M. D., and Burger, L. W. Jr. (2018). “Precision conservation to enhance wildlife benefits in agricultural landscapes,” in Precision Conservation: Geospatial Techniques for Agricultural and Natural Resources Conservation. Agronomy Monograph 59 , eds J. Delgado, G. Sassenrath, and T. Mueller (Madison, WI: ASA, CSSA, and SSSA, 285–312.
Moore, G. A. (1991). Crossing The Chasm: Marketing and Selling Technology Products to Mainstream Customers . New York, NY: Harper Business.
Noel, A. (2019). Data Becomes Cash Crop in Big Agriculture . Available online at: https://www.bloomberg.com/news/articles/2019-03-13/data-becomes-cash-crop-for-big-agriculture agriculture (accessed March 13, 2010).
Paustian, K., Easter, M., Brown, K., Chambers, A., Eve, M., Huber, A., et al. (2018). “Field- and farm-scale assessment of soil greenhouse gas mitigation using COMET-farm,” in Precision Conservation: Geospatial Techniques for Agricultural and Natural Resources Conservation. Agronomy Monograph 59 , eds J. Delgado, G. Sassenrath, and T. Mueller (Madison, WI: ASA, CSSA, and SSSA, 341–360.
Pierce, F. J., and Nowak, P. (1999). Aspects of precision agriculture. Adv. Agron. 67:1–85. doi: 10.1016/S0065-2113(08)60513-1
Pinter, P., Ritchie, J., Hatfield, J., and Hart, G. (2003). The Agricultural Research Service's remote sensing program: an example of interagency collaboration. Photogram. Engin. Remote Sensing . 69, 615–618. doi: 10.14358/PERS.69.6.615
Pruski, F. F., and Nearing, M. A. (2002). Runoff and soil loss responses to changes in precipitation: a computer simulation study. J. Soil Water Conserv . 57, 7–16.
Rabalais, N. N., Turner, N. E., and Scavia, D. (2002b). Beyond science into policy: Gulf of Mexico hypoxia and the Mississippi River. Bioscience 52, 129–142. doi: 10.1641/0006-3568(2002)052[0129:BSIPGO]2.0.CO;2
Rabalais, N. N., Turner, R. E., and Wiseman, W. J. Jr. (2002a). Gulf of Mexico hypoxia, a.k.a. The dead zone. Annu. Rev. Ecol. Evol. Syst. 33, 235–263. doi: 10.1146/annurev.ecolsys.33.010802.150513
Ribaudo, M., Delgado, J. A., Hansen, L., Livingston, M., Mosheim, R., and Williamson, J. (2011a). Nitrogen in Agricultural Systems: Implications for Conservation Policy . ERS: Economic Research Report Number 127. Washington, DC, 1–82.
Ribaudo, M., Delgado, J. A., and Livingston, M. (2011b). Preliminary assessment of the potential for nitrous oxide offsets in a cap and trade program. Agric. Resour. Econ. Rev . 40, 1–16. doi: 10.1017/S1068280500008054
Ribaudo, M. O., Heimlich, R., and Peters, M. (2005). Nitrogen sources and Gulf hypoxia: potential for environmental credit trading. Ecol. Econ . 52, 159–168. doi: 10.1016/j.ecolecon.2004.07.021
Roberts, T. L. (2007). Right product, right rate, right time, and right place…the foundation of best management practices for fertilizer, in Fertilizer Best Management Practices: General Principles, Strategies for Their Adoption, and Voluntary Initiatives vs Regulations. Paper presented at the IFA International Workshop on Fertilizer Best Management Practices, Brussels, Belgium, March 7-9, 2007 (Paris: International Fertilizer Industry Association), 29–32.
Rose, D., Sutherland, W., Parker, C., Lobbly, M., Winter, M., Morris, C., et al. (2016). Decision support tools for agriculture: towards effective design and delivery. Agric. Sys . 149, 165–174. doi: 10.1016/j.agsy.2016.09.009
Salas, W. (2010). Agricultural Strategies for Mitigating GHG Emission: DNDC Model and Case Studies . Available online at: https://ww3.arb.ca.gov/research/seminars/salas/salas.pdf
Saleh, A., and Osei, E. (2018). “Precision conservation and water quality markets,” in Precision Conservation: Geospatial Techniques for Agricultural and Natural Resources Conservation. Agronomy Monograph 59 , eds J. Delgado, G. Sassenrath, and T. Mueller (Madison, WI: ASA, CSSA, and SSSA, 313–340.
Sassenrath, G. F., and Delgado, J. A. (2018). “Precision conservation and precision regulation,” in Precision Conservation: Geospatial Techniques for Agricultural and Natural Resources Conservation , eds J. A. Delgado, G. F. Sassenrath, and T. Mueller (Madison, WI: ASA, CSSA, and SSSA).
Schimmelphfenning, D. (2016). Farm Profits and Adoption of Precision Agriculture . Available online at: https://www.ers.usda.gov/webdocs/publications/80326/err-217.pdf?v=0 , USDA ERS
Shama, J. (2017). Immediate Conditions Could Slow Adoption of Precision Agriculture, Expert Says . Available online at: https://www.agweek.com/news/4219103-immediate-conditions-could-slow-adoption-~precision-agriculture-expert-says
Shedekar, V. S., and Brown, L. C. (2018). “GIS and GPS applications for planning, design and management of drainage systems,” in Precision Conservation: Geospatial Techniques for Agricultural and Natural Resources Conservation. Agronomy Monograph 59 , eds J. Delgado, G. Sassenrath, and T. Mueller (Madison, WI: ASA, CSSA, and SSSA, 209–230.
Short, N., Cromp, R., Campbell, W., Tilton, J., LeMoigne, J., Fekete, G., et al. (1995). Mission to planet earth: AI views the world. IEEE Expert 10, 24–34. doi: 10.1109/64.483009
Short, N. Jr., and Dickens, L. (1995). Automatic generation of products from terabyte-size geographical information systems using planning/scheduling. Int. J. Geog. Inform. Sys. 9, 47–65. doi: 10.1080/02693799508902024
Sims, T. (2019). Exclusive: Microsoft Registers Blockchain and AI Platform for Agriculture in Brazil. CointTelegraph: The Future of Money . Available online at: https://cointelegraph.com/news/exclusive-microsoft-registers-blockchain-and-ai-platform-for-agriculture-in-brazil
Smith, D. R., Wilson, R. S., King, K. W., Zwonitzer, M., McGrath, J. M., Harmel, R. D., et al. (2018). Lake Erie, phosphorus, and microcystin: is it really the farmer's fault? J. Soil Water Conserv . 73, 48–57. doi: 10.2489/jswc.73.1.48
Smith, R. A., Schwarz, G. E., and Alexander, R. B. (1997). SPARROW Surface Water-Quality Modeling Nutrients in Watersheds of the Conterminous United States: Model Predictions for Total Nitrogen (TN) and Total Phosphorus (TP) . Available online at http://water.usgs.gov/nawqa/sparrow/wrr97/results.html
Spiegal, S., Bestelmeyer, B. T., Archer, D. W., Augustine, D. J., Boughton, E. H., Boughton, R. K., et al. (2018). Evaluating strategies for sustainable intensification of US agriculture through the long-term agroecosystem research network. Environ. Res. Lett . 13:034031. doi: 10.1088/1748-9326/aaa779
Steiner, J. L., Sadler, E. J., Wilson, G. J., Hatfield, J. L., James, D. E., Vandenberg, B. C., et al. (2009). STEWARDS watershed data system: system design and implementation. Trans. ASABE . 52, 1523–1533. doi: 10.13031/2013.29141
SWCS (2003). Conservation Implications of Climate Change: Soil Erosion and Runoff From Cropland. A Report From the Soil and Water Conservation Society . Ankeny, IA: Soil and Water Conservation Society.
Tayyebi, A. (2019). High Resolution Land Cover Mapping Using Deep Learning . Available online at: https://medium.com/geoai/high-resolution-land-cover-mapping-using-deep-learning-7126fee571dd
Thompson, A., and Sudduth, K. (2018). “Terracing and contour farming,” in Precision Conservation: Geospatial Techniques for Agricultural and Natural Resources Conservation. Agronomy Monograph 59 , eds J. Delgado, G. Sassenrath, and T. Mueller (Madison, WI: ASA, CSSA, and SSSA, 151–164.
Tyler, M., and Griffin, T. (2016). “Defining the barriers to telematics for precision agriculture: connectivity supply and demand,” in Southern Agricultural Economics Association 2016 Annual Meeting (San Antonio, TX). Available online at: https://ageconsearch.umn.edu/record/230090/files/Paper169.pdf
U.S. Environmental Protection Agency (2010). Inventory of U.S. Greenhouse Gas Emissions and Sinks: 1990-2008 . EPA 430-R-10-006.
United Nations (2018). Statement by the Secretary-General on the IPCC Special Report Global Warming of 1.5°C . Available online at: https://www.un.org/sg/ en/content/sg/statement/2018-10-08/ statement-secretary-general-ipcc-special-report-global-warming-15-%C2%BAc (accessed October 8, 2018).
United States Environmental Protection Agency. (2015). 2015 Drinking Water Health Advisories for Two Cyanobacterial Toxins . Washington, DC: Office of Water. 820F15003. Available online at: https://www.epa.gov/sites/production/files/2017-06/documents/cyanotoxins-fact_sheet-2015.pdf
University of New Hampshire (2012). User's Guide for the DNDC Model . Institute for the Study of Earth, Oceans, and Space.
URISA (2018). Geospatial Fact Sheet: Geospatial Data Act of 2018 . Available online at: https://www.urisa.org/clientuploads/directory/Documents/Advocacy/URISA_GeospatialFactShe~et_GeospatialDataAct_Nov2018.pdf
USDA-NRCS (2017). NRCS & Precision Conservation Management. Available online at: https://www.nrcs.usda.gov/wps/portal/nrcs/detail/?navtype=SUBNAVIGATION&ss=161017&cid=NRCSEPRD1372142&navid=105100000000000&pnavid=105000000000000&position=News&ttype=detail
USDA-NRCS (2018). Environmental Quality Incentives Program . Washington, DC: National Resources Conservation Service. Available online at: https://www.nrcs.usda.gov/wps/portal/nrcs/main/national/programs/financial/eqip/
Van der Wal, T. (2019). Why is Adoption of Precision ag so Slow? Available online at: https://www.futurefarming.com/Smart-farmers/Articles/2019/1/Why-is-adoption-of-precision-~ag-so-slow-385338E/
Vasisht, D.l, Kapetanovic, Z., Won, J., Jin, X., Chandra, R., Kapoor, A., Sinha, S., et al. (2017). “FarmBeats: an IoT platform for data-driven agriculture,” in Proceedings of the 14 th USENIX Symposium on Networked Systems Design and Implementation (NSDI'17), March 2017 (Boston, MA).
Verdouw, C., and Kruize, J. (2015). “Digital twins in farm management: illustrations from the FIWARE accelerators SmartAgriFood and Fractals,” in 7th Asian-Australasian Conference on Precision Agriculture, 2015 (Hamilton: Precision Agriculture Association New Zealand).
Vitousek, P. M., Aber, J. D., Howarth, R. W., Likens, G. E., Matson, P. A., Schindler, D. W., et al. (1997). Human alteration of the global nitrogen cycle: sources and consequences. Ecol. Appl. 73, 737–750. doi: 10.1890/1051-0761(1997)007[0737:HAOTGN]2.0.CO;2
Walthall, C. L., Hatfield, J., Backlund, P., Lengnick, L., Marshall, E., Walsh, M., et al. (2012). Climate Change and Agriculture in the United States: Effects and Adaptation . USDA Technical Bulletin 1935. Washington, DC: USDA.
Wolfert, S., Cor Verdouw, L., and Bogaardt, M. (2017). Big data in smart farming – A review. Agricult. Syst. 153, 69–80. doi: 10.1016/j.agsy.2017.01.023
World Health Organization (2011). Guidelines for Drinking-water Quality, 4th Edn . Geneva: World Health Organization Press.
Keywords: big data, analytics, remote sensing—GIS, artificial intelligence, precision agriculture, sustainable agriculture
Citation: Delgado JA, Short NM Jr, Roberts DP and Vandenberg B (2019) Big Data Analysis for Sustainable Agriculture on a Geospatial Cloud Framework. Front. Sustain. Food Syst. 3:54. doi: 10.3389/fsufs.2019.00054
Received: 30 April 2019; Accepted: 27 June 2019; Published: 16 July 2019.
Reviewed by:
Copyright © 2019 Delgado, Short, Roberts and Vandenberg. This is an open-access article distributed under the terms of the Creative Commons Attribution License (CC BY) . The use, distribution or reproduction in other forums is permitted, provided the original author(s) and the copyright owner(s) are credited and that the original publication in this journal is cited, in accordance with accepted academic practice. No use, distribution or reproduction is permitted which does not comply with these terms.
*Correspondence: Nicholas M. Short Jr., nshort@esri.com
This article is part of the Research Topic
Sustainable Production of Nutrition-Dense Crops
Essay on Sustainable Agriculture
Introduction: what is sustainable agriculture, importance of sustainable agriculture, population growth, per capita food consumption, sustainable agriculture and technology, green politics, conclusion of sustainable agriculture.
Bibliography
Sustainable agriculture has dominated the sociological understanding of the rural world largely. Following the enthusiasm around the concept as a means of eradication of poverty and turning the economy to a “resource-efficient, low carbon Green Economy” 1 . Global population, and consequently consumption has increased.
However, technology development has matched the demand for food in terms of food production, but the distribution of food is not evenly distributed. This has brought forth the question of the possibility of supplying adequate food to the ever-growing global population.
Further, the challenges posed by depleting non-renewable sources of energy, rising costs, and climate change has brought the issue related to sustainability of food production and the related social and economic impact of the food production into forefront. This paper outlines the meaning and technology related to sustainable agriculture and tries to gauge its impact as a possible solution to the impending food crisis.
Sustainable agriculture is a process of farming using eco-friendly methods understanding and maintaining the relationship between the organisms and environment. In this process of agriculture and animal husbandry are combined to form a simultaneous process and practice. In other words, sustainable agriculture is an amalgamation of three main elements viz. ecological health, profitability, and propagating equality.
The concept of sustainability rests on the principle of not wasting any resources that may become useful to the future generation. Therefore, the main idea of sustainability rests on stewardship of individual and natural resources. Before understanding the technology involved in sustainable agriculture, it is important to know why we need it in the first place.
The rise in population growth and urbanization of people has led to a dietary change of the world population, which now rests more on animal protein 2 . Therefore understanding the demographic changes in the world population has become an important parameter to judge the future demand for food.
As population growth rate is the key variable that affects the demand for food, therefore understanding the number of people increasing worldwide is important. According to the UNDP results, the annual population growth rate had declined from 2.2% in 1962 to 1.1% in 2010, however, this increase to indicate an increase of 75 million people 3 .
However, this increase in population is not equitably distributed as some areas such as Africa, Latin America, and Asia face a growth rate of 2% while others such as the erstwhile Soviet bloc countries have a negative rate.
According to the UNDP predictions, population worldwide is expected to increase to 9 billion in 2050 from the present 7 billion 4 . Therefore, the uncertain growth in population is expected to affect food demand and therefore food production.
Undernourishment is a prevalent problem in the developing world, wherein almost 20% of the developing world that is more than 5 billion people is undernourished.
Further, in emerging economies, food consumption is increasing with increased preference for animal protein such as meat, dairy products, and egg. Therefore, the growth of consumption of animal protein has increased the necessity of grazing of livestock, therefore, increasing further pressure on the food supply.
It is believed that the increase in the demand for food due to the increase in global population and change in dietary habit of the population. In the past, the demand for food and the rate of production has remained at par, but the unequal distribution of food has led to the major problem in food supply and starvation in various parts of the world.
Another problem that food production in the future faces is the constraint of non-renewable natural resources. The most critical resources, which are becoming scant for the future generations are –
- Land : Availability of land globally to cultivate food has grown marginally due to the increase in global population. The availability of land available per person to grow food has declined from 1.30 hectares in 1967 to 0.72 hectares in 2007 5 . Therefore, a clear dearth in agricultural land is a deterrent to future agriculture.
- Water : The world comprises of 70% freshwater resources, available from river and groundwater. Deficiency of freshwater has been growing as usage of water has increased more than twice the rate of population growth 6 . As water is required for irrigation purposes, water availability to is not equally distributed around the world. Therefore, reduced water supply would limit the per capita production of food.
- Energy : Globally, the scarcity of the non-renewable resources of energy is another concern. The global demand for energy is expected to double by 2050, consequently increasing energy prices 7 . Therefore, food production for the future will have to devise a technology based on renewable sources of energy.
The question of sustainability in agriculture arose due to some pressing issues that have limited the utilization of erstwhile processes and technologies for food production. However, it should be noted that sustainable agriculture does not prescribe any set rule or technology for the production process, rather shows a way towards sustainability 8 .
Sustainable agriculture uses best management practice by adhering to target-oriented cultivation. The agriculture process looks at disease-oriented hybrid, pest control through use of biological insecticides and low usage of chemical pesticide and fertilizer. Usually, insect-specific pest control is used, which is biological in nature.
Water given to the crops is through micro-sprinklers which help is directly watering the roots of the plants, and not flooding the field completely. The idea is to manage the agricultural land for both plants and animal husbandry.
For instance, in many southwestern parts of Florida’s citrus orchards, areas meant for water retention and forest areas become a natural habitat for birds and other animals 9 . The process uses integrated pest management that helps in reducing the amount of pesticide used in cultivation.
Sustainable agriculture adopts green technology as a means of reducing wastage of non-renewable energy and increase production. In this respect, the sustainable agricultural technology is linked to the overall developmental objective of the nation and is directly related to solving socio-economic problems of the nation 10 .
The UN report states, “The productivity increases in possible through environment-friendly and profitable technologies.” 11 In order to understand the technology better, one must realize that the soil’s health is crucial for cultivation of crops.
Soil is not just another ingredient for cultivation like pesticides or fertilizers; rather, it is a complex and fragile medium that must be nurtured to ensure higher productivity 12 . Therefore, the health of the soil can be maintained using eco-friendly methods:
Healthy soil, essential to agriculture, is a complex, living medium. The loose but coherent structure of good soil holds moisture and invites airflow. Ants (a) and earthworms (b) mix the soil naturally. Rhizobium bacteria (c) living in the root nodules of legumes (such as soybeans) create fixed nitrogen, an essential plant nutrient.
Other soil microorganisms, including fungi (d), actinomycetes (e) and bacteria (f), decompose organic matter, thereby releasing more nutrients. Microorganisms also produce substances that help soil particles adhere to one another. To remain healthy, soil must be fed organic materials such as various manures and crop residues. 13
This is nothing but a broader term to denote environment-friendly solutions to agricultural production. Therefore, the technology-related issue of sustainable agriculture is that it should use such technology that allows usage of renewable sources of energy and is not deterrent to the overall environment.
The politics around sustainable agriculture lies in the usage of the renewable sources of energy and disciplining of the current consumption rates 14 . The politics related to the sustainable agriculture is also related to the politics of sustainable consumption.
Though there is a growing concern over depleting food for the future and other resources, there is hardly any measure imposed by the governments of developed and emerging economies to sustain the consumption pattern of the population 15 .
The advocates of green politics believe that a radical change of the conventional agricultural process is required for bringing forth sustainable agriculture 16 . Green politics lobbies for an integrated farming system that can be the only way to usher in sustainable agricultural program 17 .
Sustainable agriculture is the way to maintain a parity between the increasing pressure of food demand and food production in the future. As population growth, change in income demographics, and food preference changes, there are changes in the demand of food of the future population.
Further, changes in climate and increasing concern regarding the depletion of non-renewable sources of energy has forced policymakers and scientists to device another way to sustain the available resources as well as continue meeting the increased demand of food.
Sustainable agriculture is the method through which these problems can be overlooked, bringing forth a new integrated form of agriculture that looks at food production in a holistic way.
Batie, S. S., ‘Sustainable Development: Challenges to Profession of Agricultural Economics’, American Journal of Agricultural Economics, vol. 71, no. 5, 1989: 1083-1101.
Dobson, A., The Politics of Nature: Explorations in Green Political Theory, Psychology Press, London, 1993.
Leaver, J. D., ‘Global food supply: a challenge for sustainable agriculture’, Nutrition Bulletin, vol. 36 , 2011: 416-421.
Martens, S., & G. Spaargaren, ‘The politics of sustainable consumption: the case of the Netherlands’, Sustainability: Science, Practice, & Policy, vol.1 no. 1, 2005: 29-42.
Morris, C., & M. Winter, ‘Integrated farming systems: the third way for European agriculture?’, Land Use Policy, vol. 16, no. 4, 1999: 193–205.
Reganold, J. P., R. I. Papendick, & J. F. Parr, ‘Sustainable Agriculture’, Scientific American , 1990: 112-120.
Townsend, C., ‘ Technology for Sustainable Agriculture. ‘ Florida Gulf Coast University, 1998. Web.
United Nations, ‘ Green technology for sustainable agriculture development ‘, United Nations Asian And Pacific Centre For Agricultural Engineering And Machinery, 2010. Web.
—, ‘ Sustainable agriculture key to green growth, poverty reduction – UN officials ‘, United Nations, 2011. Web.
1 United Nations, Sustainable agriculture key to green growth, poverty reduction – UN officials, UN News Centre, 2011.
2 J. D. Leaver, ‘Global food supply: a challenge for sustainable agriculture’, Nutrition Bulletin , vol. 36, 2011, pp. 416-421.
3 Leaver, p. 417.
5 Leaver, p. 418.
7 Leaver, p. 419.
8 J. N. Pretty, ‘Participatory learning for sustainable agriculture’, World Development , vol. 23, no. 8, 1995, pp. 1247-1263.
9 Chet Townsend, ‘Technology for Sustainable Agriculture’, Florida Gulf Coast University , 1998.
10 United Nations, ‘Green technology for sustainable agriculture development’, United Nations Asian And Pacific Centre For Agricultural Engineering And Machinery , 2010.
11 United Nations, p. 17.
12 J. P. Reganold, R. I. Papendick, & J. F. Parr, ‘Sustainable Agriculture’, Scientific American , 1990, pp. 112-120.
13 Regnold et al., p. 112.
14 S. S. Batie, ‘Sustainable Development: Challenges to Profession of Agricultural Economics’, American Journal of Agricultural Economics, vol. 71, no. 5, 1989, pp. 1083-1101.
15 S. Martens & G. Spaargaren, ‘The politics of sustainable consumption: the case of the Netherlands’, Sustainability: Science, Practice, & Policy , vol.1 no. 1, 2005, pp. 29-42.
16 A. Dobson, The Politics of Nature: Explorations in Green Political Theory , Psychology Press, London, 1993, p. 82.
17 C .Morris & M. Winter, ‘Integrated farming systems: the third way for European agriculture?’, Land Use Policy , vol. 16, no. 4, 1999, pp. 193–205.
- Chicago (A-D)
- Chicago (N-B)
IvyPanda. (2019, April 20). Essay on Sustainable Agriculture. https://ivypanda.com/essays/sustainable-agriculture-essay/
"Essay on Sustainable Agriculture." IvyPanda , 20 Apr. 2019, ivypanda.com/essays/sustainable-agriculture-essay/.
IvyPanda . (2019) 'Essay on Sustainable Agriculture'. 20 April.
IvyPanda . 2019. "Essay on Sustainable Agriculture." April 20, 2019. https://ivypanda.com/essays/sustainable-agriculture-essay/.
1. IvyPanda . "Essay on Sustainable Agriculture." April 20, 2019. https://ivypanda.com/essays/sustainable-agriculture-essay/.
IvyPanda . "Essay on Sustainable Agriculture." April 20, 2019. https://ivypanda.com/essays/sustainable-agriculture-essay/.
- Natural Resources, Their Classification and Impacts
- Smart Farming and Sustainable Agriculture
- Cannabis Technological Advancement in Cultivation
- Irrigation System Strategy
- Irrigation Systems in Farming
- Swidden Agriculture: Shift Farming
- Whaling in Japan: Justifiable by Culture?
- Essential Foods Price: Basics Foods and High Increase in Prices
- MyU : For Students, Faculty, and Staff
News Roundup Spring 2024
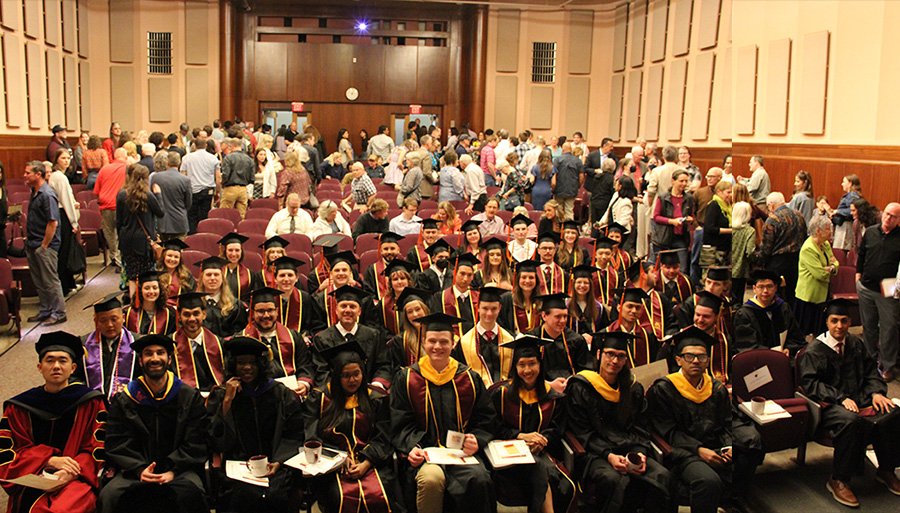
CEGE Spring Graduation Celebration and Order of the Engineer
Forty-seven graduates of the undergraduate and grad student programs (pictured above) in the Department of Civil, Environmental, and Geo- Engineering took part in the Order of the Engineer on graduation day. Distinguished Speakers at this departmental event included Katrina Kessler (MS EnvE 2021), Commissioner of the Minnesota Pollution Control Agency, and student Brian Balquist. Following this event, students participated in the college-wide Commencement Ceremony at 3M Arena at Mariucci.
UNIVERSITY & DEPARTMENT
The University of Minnesota’s Crookston, Duluth, and Rochester campuses have been awarded the Carnegie Elective Classification for Community Engagement, joining the Twin Cities (2006, 2015) and Morris campuses (2015), and making the U of M the country’s first and only university system at which every individual campus has received this selective designation. Only 368 from nearly 4,000 qualifying U.S. universities and colleges have been granted this designation.
CEGE contributed strongly to the College of Science and Engineering’s efforts toward sustainability research. CEGE researchers are bringing in over $35 million in funded research to study carbon mineralization, nature and urban areas, circularity of water resources, and global snowfall patterns. This news was highlighted in the Fall 2023 issue of Inventing Tomorrow (pages 10-11). https://issuu.com/inventingtomorrow/docs/fall_2023_inventing_tomorrow-web
CEGE’s new program for a one-year master’s degree in structural engineering is now accepting applicants for Fall 2024. We owe a big thanks to DAN MURPHY and LAURA AMUNDSON for their volunteer work to help curate the program with Professor JIA-LIANG LE and EBRAHIM SHEMSHADIAN, the program director. Potential students and companies interested in hosting a summer intern can contact Ebrahim Shemshadian ( [email protected] ).
BERNIE BULLERT , CEGE benefactor and MN Water Research Fund founder, was profiled on the website of the University of Minnesota Foundation (UMF). There you can read more about his mission to share clean water technologies with smaller communities in Minnesota. Many have joined Bullert in this mission. MWRF Recognizes their Generous 2024 Partners. Gold Partners: Bernie Bullert, Hawkins, Inc., Minnesota Department of Health, Minnesota Pollution Control Agency, and SL-serco. Silver Partners: ISG, Karl and Pam Streed, Kasco, Kelly Lange-Haider and Mark Haider, ME Simpson, Naeem Qureshi, Dr. Paul H. Boening, TKDA, and Waterous. Bronze Partners: Bruce R. Bullert; Brenda Lenz, Ph.D., APRN FNP-C, CNE; CDM Smith; Central States Water Environment Association (CSWEA MN); Heidi and Steve Hamilton; Jim “Bulldog” Sadler; Lisa and Del Cerney; Magney Construction; Sambatek; Shannon and John Wolkerstorfer; Stantec; and Tenon Systems.
After retiring from Baker-Tilly, NICK DRAGISICH (BCE 1977) has taken on a new role: City Council member in Lake Elmo, Minnesota. After earning his BCE from the University of Minnesota, Dragisich earned a master’s degree in business administration from the University of St. Thomas. Dragisich retired in May from his position as managing director at Baker Tilly, where he had previously served as firm director. Prior to that, he served as assistant city manager in Spokane, Washington, was the city administrator and city engineer in Virginia, Minnesota, and was mayor of Chisholm, Minnesota—all adding up to more than 40 years of experience in local government. Dragisich was selected by a unanimous vote. His current term expires in December 2024.
PAUL F. GNIRK (Ph.D. 1966) passed away January 29, 2024, at the age of 86. A memorial service was held Saturday, February 24, at the South Dakota School of Mines and Technology (SDSM&T), where he started and ended his teaching career, though he had many other positions, professional and voluntary. In 2018 Paul was inducted into the SDSM&T Hardrocker Hall of Fame, and in 2022, he was inducted into the South Dakota Hall of Fame, joining his mother Adeline S. Gnirk, who had been inducted in 1987 for her work authoring nine books on the history of south central South Dakota.
ROGER M. HILL (BCE 1957) passed away on January 13, 2024, at the age of 90. His daughter, Kelly Robinson, wrote to CEGE that Roger was “a dedicated Gopher fan until the end, and we enjoyed many football games together in recent years. Thank you for everything.”
KAUSER JAHAN (Ph.D. 1993, advised by Walter Maier), PE, is now a civil and environmental engineering professor and department head at Henry M. Rowan College of Engineering. Jahan was awarded a 3-year (2022- 2025), $500,000 grant from the U.S. Department of Environmental Protection Agency (USEPA). The grant supports her project, “WaterWorks: Developing the New Generation of Workforce for Water/Wastewater Utilities,” for the development of educational tools that will expose and prepare today’s students for careers in water and wastewater utilities.
SAURA JOST (BCE 2010, advised by Timothy LaPara) was elected to the St. Paul City Council for Ward 3. She is part of the historic group of women that make up the nation’s first all-female city council in a large city.
The 2024 ASCE Western Great Lakes Student Symposium combines several competitions for students involved in ASCE. CEGE sent a large contingent of competitors to Chicago. Each of the competition groups won awards: Ethics Paper 1st place Hans Lagerquist; Sustainable Solutions team 1st place overall in (qualifying them for the National competition in Utah in June); GeoWall 2nd place overall; Men’s Sprint for Concrete Canoe with rowers Sakthi Sundaram Saravanan and Owen McDonald 2nd place; Product Prototype for Concrete Canoe 2nd place; Steel Bridge (200 lb bridge weight) 2nd place in lightness; Scavenger Hunt 3rd place; and Aesthetics and Structural Efficiency for Steel Bridge 4th place.
Students competing on the Minnesota Environmental Engineers, Scientists, and Enthusiasts (MEESE) team earned second place in the Conference on the Environment undergraduate student design competition in November 2023. Erin Surdo is the MEESE Faculty Adviser. Pictured are NIKO DESHPANDE, ANNA RETTLER, and SYDNEY OLSON.
The CEGE CLASS OF 2023 raised money to help reduce the financial barrier for fellow students taking the Fundamentals of Engineering exam, a cost of $175 per test taker. As a result of this gift, they were able to make the exam more affordable for 15 current CEGE seniors. CEGE students who take the FE exam pass the first time at a rate well above national averages, demonstrating that CEGE does a great job of teaching engineering fundamentals. In 2023, 46 of 50 students passed the challenging exam on the first try.
This winter break, four CEGE students joined 10 other students from the College of Science and Engineering for the global seminar, Design for Life: Water in Tanzania. The students visited numerous sites in Tanzania, collected water source samples, designed rural water systems, and went on safari. Read the trip blog: http://globalblogs.cse.umn.edu/search/label/Tanzania%202024
Undergraduate Honor Student MALIK KHADAR (advised by Dr. Paul Capel) received honorable mention for the Computing Research Association (CRA) Outstanding Undergraduate Research Award for undergraduate students who show outstanding research potential in an area of computing research.
GRADUATE STUDENTS
AKASH BHAT (advised by William Arnold) presented his Ph.D. defense on Friday, October 27, 2023. Bhat’s thesis is “Photolysis of fluorochemicals: Tracking fluorine, use of UV-LEDs, and computational insights.” Bhat’s work investigating the degradation of fluorinated compounds will assist in the future design of fluorinated chemicals such that persistent and/or toxic byproducts are not formed in the environment.
ETHAN BOTMEN (advised by Bill Arnold) completed his Master of Science Final Exam February 28, 2024. His research topic was Degradation of Fluorinated Compounds by Nucleophilic Attack of Organo-fluorine Functional Groups.
XIATING CHEN , Ph.D. Candidate in Water Resources Engineering at the Saint Anthony Falls Laboratory is the recipient of the 2023 Nels Nelson Memorial Fellowship Award. Chen (advised by Xue Feng) is researching eco-hydrological functions of urban trees and other green infrastructure at both the local and watershed scale, through combined field observations and modeling approaches.
ALICE PRATES BISSO DAMBROZ has been a Visiting Student Researcher at the University of Minnesota since last August, on a Doctoral Dissertation Research Award from Fulbright. Her CEGE advisor is Dr. Paul Capel. Dambroz is a fourth year Ph.D. student in Soil Science at Universidade Federal de Santa Maria in Brazil, where she studies with her adviser Jean Minella. Her research focuses on the hydrological monitoring of a small agricultural watershed in Southern Brazil, which is located on a transition area between volcanic and sedimentary rocks. Its topography, shallow soils, and land use make it prone to runoff and erosion processes.
Yielding to people in crosswalks should be a very pedestrian topic. Yet graduate student researchers TIANYI LI, JOSHUA KLAVINS, TE XU, NIAZ MAHMUD ZAFRI (Dept.of Urban and Regional Planning at Bangladesh University of Engineering and Technology), and Professor Raphael Stern found that drivers often do not yield to pedestrians, but they are influenced by the markings around a crosswalk. Their work was picked up by the Minnesota Reformer.
TIANYI LI (Ph.D. student advised by Raphael Stern) also won the Dwight David Eisenhower Transportation (DDET) Fellowship for the third time! Li (center) and Stern (right) are pictured at the Federal Highway Administration with Latoya Jones, the program manager for the DDET Fellowship.
The Three Minute Thesis Contest and the Minnesota Nice trophy has become an annual tradition in CEGE. 2023’s winner was EHSANUR RAHMAN , a Ph.D. student advised by Boya Xiong.
GUANJU (WILLIAM) WEI , a Ph.D. student advised by Judy Yang, is the recipient of the 2023 Heinz G. Stefan Fellowship. He presented his research entitled Microfluidic Investigation of the Biofilm Growth under Dynamic Fluid Environments and received his award at the St. Anthony Falls Research Laboratory April 9. The results of Wei's research can be used in industrial, medical, and scientific fields to control biofilm growth.
BILL ARNOLD stars in an award-winning video about prairie potholes. The Prairie Potholes Project film was made with the University of Delaware and highlights Arnold’s NSF research. The official winners of the 2024 Environmental Communications Awards Competition Grand Prize are Jon Cox and Ben Hemmings who produced and directed the film. Graduate student Marcia Pacheco (CFANS/LAAS) and Bill Arnold are the on-screen stars.
Four faculty from CEGE join the Center for Transportation Studies Faculty and Research Scholars for FY24–25: SEONGJIN CHOI, KETSON ROBERTO MAXIMIANO DOS SANTOS, PEDRAM MORTAZAVI, and BENJAMIN WORSFOLD . CTS Scholars are drawn from diverse fields including engineering, planning, computer science, environmental studies, and public policy.
XUE FENG is coauthor on an article in Nature Reviews Earth and Environment . The authors evaluate global plant responses to changing rainfall regimes that are now characterized by fewer and larger rainfall events. A news release written at Univ. of Maryland can be found here: https://webhost.essic. umd.edu/april-showers-bring-mayflowers- but-with-drizzles-or-downpours/ A long-running series of U of M research projects aimed at improving stormwater quality are beginning to see practical application by stormwater specialists from the Twin Cities metro area and beyond. JOHN GULLIVER has been studying best practices for stormwater management for about 16 years. Lately, he has focused specifically on mitigating phosphorous contamination. His research was highlighted by the Center for Transportation Studies.
JIAQI LI, BILL ARNOLD, and RAYMOND HOZALSKI published a paper on N-nitrosodimethylamine (NDMA) precursors in Minnesota rivers. “Animal Feedlots and Domestic Wastewater Discharges are Likely Sources of N-Nitrosodimethylamine (NDMA) Precursors in Midwestern Watersheds,” Environmental Science and Technology (January 2024) doi: 10.1021/acs. est.3c09251
ALIREZA KHANI contributed to MnDOT research on Optimizing Charging Infrastructure for Electric Trucks. Electric options for medium- and heavy-duty electric trucks (e-trucks) are still largely in development. These trucks account for a substantial percentage of transportation greenhouse gas emissions. They have greater power needs and different charging needs than personal EVs. Proactively planning for e-truck charging stations will support MnDOT in helping to achieve the state’s greenhouse gas reduction goals. This research was featured in the webinar “Electrification of the Freight System in Minnesota,” hosted by the University of Minnesota’s Center for Transportation Studies. A recording of the event is now available online.
MICHAEL LEVIN has developed a unique course for CEGE students on Air Transportation Systems. It is the only class at UMN studying air transportation systems from an infrastructure design and management perspective. Spring 2024 saw the third offering of this course, which is offered for juniors, seniors, and graduate students.
Research Professor SOFIA (SONIA) MOGILEVSKAYA has been developing international connections. She visited the University of Seville, Spain, November 13–26, 2023, where she taught a short course titled “Fundamentals of Homogenization in Composites.” She also met with the graduate students to discuss collaborative research with Prof. Vladislav Mantic, from the Group of Continuum Mechanics and Structural Analysis at the University of Seville. Her visit was a part of planned activities within the DIAGONAL Consortium funded by the European Commission. CEGE UMN is a partner organization within DIAGONAL, represented by CEGE professors Mogilevskaya and Joseph Labuz. Mantic will visit CEGE summer 2024 to follow up on research developments and discuss plans for future collaboration and organization of short-term exchange visits for the graduate students from each institution.
DAVID NEWCOMB passed away in March. He was a professor in CEGE from 1989–99 in the area of pavement engineering. Newcomb led the research program on asphalt materials characterization. He was the technical director of Mn/ROAD pavement research facility, and he started an enduring collaboration with MnDOT that continues today. In 2000, he moved from Minnesota to become vice-president for Research and Technology at the National Asphalt Pavement Association. Later he moved to his native Texas, where he was appointed to the division head of Materials and Pavement at the Texas A&M Transportation Institute, a position from which he recently retired. He will be greatly missed.
PAIGE NOVAK won Minnesota ASCE’s 2023 Distinguished Engineer of the Year Award for her contributions to society through her engineering achievements and professional experiences.
The National Science Foundation (NSF) announced ten inaugural (NSF) Regional Innovation Engines awards, with a potential $1.6 billion investment nationally over the next decade. Great Lakes ReNEW is led by the Chicago-based water innovation hub, Current, and includes a team from the University of Minnesota, including PAIGE NOVAK. Current will receive $15 mil for the first two years, and up to $160 million over ten years to develop and grow a water-focused innovation engine in the Great Lakes region. The project’s ambitious plan is to create a decarbonized circular “blue economy” to leverage the region’s extraordinary water resources to transform the upper Midwest—Illinois, Indiana, Michigan, Minnesota, Ohio, and Wisconsin. Brewing one pint of beer generates seven pints of wastewater, on average. So what can you do with that wastewater? PAIGE NOVAK and her team are exploring the possibilities of capturing pollutants in wastewater and using bacteria to transform them into energy.
BOYA XIONG has been selected as a recipient of the 2024 40 Under 40 Recognition Program by the American Academy of Environmental Engineers and Scientists. The award was presented at the 2024 AAEES Awards Ceremony, April 11, 2024, at the historic Howard University in Washington, D.C.
JUDY Q. YANG received a McKnight Land-Grant Professorship Award. This two-year award recognizes promising assistant professors and is intended to advance the careers of individuals who have the potential to make significant contributions to their departments and their scholarly fields.
Professor Emeritus CHARLES FAIRHURST , his son CHARLES EDWARD FAIRHURST , and his daughter MARGARET FAIRHURST DURENBERGER were on campus recently to present Department Head Paige Novak with a check for $25,000 for the Charles Fairhurst Fellowship in Earth Resources Engineering in support of graduate students studying geomechanics. The life of Charles Fairhurst through a discussion with his children is featured on the Engineering and Technology History Wiki at https://ethw.org/Oral-History:Charles_Fairhurst#00:00:14_INTRODUCTION
Related news releases
- Matthew J. Huber Student Award
- Catherine French, NAE
- Climate Change for Engineers
- Focused on the Road Ahead
- Randal Barnes receives Horace T Morace Award
- Future undergraduate students
- Future transfer students
- Future graduate students
- Future international students
- Diversity and Inclusion Opportunities
- Learn abroad
- Living Learning Communities
- Mentor programs
- Programs for women
- Student groups
- Visit, Apply & Next Steps
- Information for current students
- Departments and majors overview
- Departments
- Undergraduate majors
- Graduate programs
- Integrated Degree Programs
- Additional degree-granting programs
- Online learning
- Academic Advising overview
- Academic Advising FAQ
- Academic Advising Blog
- Appointments and drop-ins
- Academic support
- Commencement
- Four-year plans
- Honors advising
- Policies, procedures, and forms
- Career Services overview
- Resumes and cover letters
- Jobs and internships
- Interviews and job offers
- CSE Career Fair
- Major and career exploration
- Graduate school
- Collegiate Life overview
- Scholarships
- Diversity & Inclusivity Alliance
- Anderson Student Innovation Labs
- Information for alumni
- Get engaged with CSE
- Upcoming events
- CSE Alumni Society Board
- Alumni volunteer interest form
- Golden Medallion Society Reunion
- 50-Year Reunion
- Alumni honors and awards
- Outstanding Achievement
- Alumni Service
- Distinguished Leadership
- Honorary Doctorate Degrees
- Nobel Laureates
- Alumni resources
- Alumni career resources
- Alumni news outlets
- CSE branded clothing
- International alumni resources
- Inventing Tomorrow magazine
- Update your info
- CSE giving overview
- Why give to CSE?
- College priorities
- Give online now
- External relations
- Giving priorities
- CSE Dean's Club
- Donor stories
- Impact of giving
- Ways to give to CSE
- Matching gifts
- CSE directories
- Invest in your company and the future
- Recruit our students
- Connect with researchers
- K-12 initiatives
- Diversity initiatives
- Research news
- Give to CSE
- CSE priorities
- Corporate relations
- Information for faculty and staff
- Administrative offices overview
- Office of the Dean
- Academic affairs
- Finance and Operations
- Communications
- Human resources
- Undergraduate programs and student services
- CSE Committees
- CSE policies overview
- Academic policies
- Faculty hiring and tenure policies
- Finance policies and information
- Graduate education policies
- Human resources policies
- Research policies
- Research overview
- Research centers and facilities
- Research proposal submission process
- Research safety
- Award-winning CSE faculty
- National academies
- University awards
- Honorary professorships
- Collegiate awards
- Other CSE honors and awards
- Staff awards
- Performance Management Process
- Work. With Flexibility in CSE
- K-12 outreach overview
- Summer camps
- Outreach events
- Enrichment programs
- Field trips and tours
- CSE K-12 Virtual Classroom Resources
- Educator development
- Sponsor an event

IMAGES
VIDEO
COMMENTS
Tel.: +49-4131-677-1582. Academic Editor: Marc A. Rosen. Received: 5 March 2015 / Accepted: 10 June 2015 / Published: 18 June 2015. Abstract: The idea of a sustainable agriculture has gained ...
Sustainable agriculture is a broad term for growing food using methods that will nurture society, the environment, and the economy. Sustainable farmers seek to support community health and well-being and to work with nature, while still being profitable businesses as well as functioning as non-profits or recreational projects. ... Thesis (2012 ...
Sustainable agriculture primarily addresses on-farm practices and systems, seeking to optimize agricultural productivity while minimizing negative impacts. Contrarily, sustainability fueled by agriculture acknowledges that the agricultural sector's actions can have significant effects on global sustainability, encompassing aspects of the ...
Sustainable agriculture outcomes can be positive for food productivity, reduced pesticide use and carbon balances. Significant challenges, however, remain to develop national and international policies to support the wider emergence of more sustainable forms of agricultural production across both industrialized and developing countries.
The adoption of sustainable agricultural practices (SAPs) has been recommended by many experts and international institutions to address food security and climate change problems. Global support for the Sustainable Development Goals has focused attention on efforts to up-scale the adoption of SAPs in developing countries where growth in populations and incomes compromises the resilience of ...
The impact of climate change on agricultural practices is raising question marks on future food security of billions of people in tropical and subtropical regions. Recently introduced, climate-smart agriculture (CSA) techniques encourage the practices of sustainable agriculture, increasing adaptive capacity and resilience to shocks at multiple levels. However, it is extremely difficult to ...
Sustainable agriculture. Nature Sustainability 1 , 531 ( 2018) Cite this article. Achieving food security is possible, if we better understand the complexity of the agricultural system and re ...
Sustainable agriculture ¡ t ¡ y ¡ ture. Dissertations in Physical Geography No. 16. Doctoral Thesis in Physical Geography at Stockholm University, Sweden 2021. Department of Physical Geography ․ y. n Environmental Science from the University of, m. g. e n the resources on which the sector depends. Opportunities for the g s
Sustainable agriculture seeks to balance human nutritional needs with the preservation of environmental quality and the economic viability of agricultural systems. Principles of resource conservation, economic resilience, social equity, and competitiveness underpin this approach. Despite its potential to address crucial issues like food security, energy sustainability, and environmental ...
1. Introduction. The agriculture sector in sub-Saharan Africa (SSA), which is the region's economic juggernaut and is primarily dominated by smallholder farmers, who account for 65% of the total household population in the region, is increasingly experiencing severe productivity decline as a result of global climate change (AGRA, 2020; Nhemachena and Panco, 2021).
The general aim of this thesis was to develop a rationale for balancing the level of context-specificity and -generality of sustainability assessment frameworks in order to optimise these tradeoffs and hence effectively and efficiently assess and incentivise the sustainability of agricultural systems.
1. Introduction. Over the past decade, there have been several efforts to promote sustainable intensification of smallholder farming systems (Barnes, Lucas, & Maio, Citation 2016; Mutyasira et al., Citation 2018a).Several sustainable agricultural practices (SAPs), including conservation tillage, soil and water conservation, legume crop rotations, improved seed varieties and use of animal ...
This thesis does not contain text, graphics or tables copied and pasted from the Internet, unless specifically acknowledged, and the source being detailed in the ... 2.10 Sustainable agriculture as an alternative to conventional agriculture 38 2.11 Practice of sustainable agriculture in South Africa 44
Furthermore, this final thesis gains relevance due to a rather weak evidence base on the role of agriculture for development and its largely disputed nature. Moreover, how agriculture evolves and adapts determines to a large degree how sustainable In-dia's and the world's future will be.
There are numerous studies and publications about sustainable agriculture. Many papers argue that sustainable agriculture is necessary, and analyze how this goal could be achieved. At the same time, studies question the sustainability of agriculture. Several obstacles, including theoretical, methodological, personal, and practical issues, hinder or slow down implementation, resulting in the so ...
1. Theories and Methods of Agricultural Sustainable Development. Food supply is one of the important purposes of sustainable agricultural development. Gilmar et al. [ 1] used an innovative in vitro technique to increase the cultivation of micro tubers, improve the yield and quality of seeds and crops, and ensure regional food supply.
Big Data Analysis for Sustainable Agriculture on a Geospatial Cloud Framework. Jorge A. Delgado 1 Nicholas M. Short Jr. 2* Daniel P. Roberts 3 Bruce Vandenberg 4. 1 Soil Management and Sugar Beet Research Unit, Agricultural Research Service, United States Department of Agriculture (USDA-ARS), Fort Collins, CO, United States.
Thesis Statement: Sustainable agriculture, while differing in its application, represents a logical, realistic, and necessary alternative to industrial agriculture given the reality of limited resources and anticipated food shortages in the 21 st century . I. Introduction. A. ...
The concepts, 'sustainable development' and 'sustainable agriculture', have been shaped by both political discourses of the United Nations and scientific discourses. Using critical discourse and content analysis, we trace the meaning of 'sustainable agriculture' in both international political and in scientific discourses to examine and identify key elements of the representation ...
Essays on Agriculture and Rural Development in Developing Countries Abstract In these three essays, I analyse the effects of institutions on rural development through the lens of natural resource management in chapter 1, agricultural productivity in chapter 2, and rural agglomeration economies in chapter 3. In chapter 1, we study whether the
Sustainable agriculture has dominated the sociological understanding of the rural world largely. Following the enthusiasm around the concept as a means of eradication of poverty and turning the economy to a "resource-efficient, low carbon Green Economy" 1. Global population, and consequently consumption has increased.
CEGE Spring Graduation Celebration and Order of the EngineerForty-seven graduates of the undergraduate and grad student programs (pictured above) in the Department of Civil, Environmental, and Geo- Engineering took part in the Order of the Engineer on graduation day. Distinguished Speakers at this departmental event included Katrina Kessler (MS EnvE 2021), Commissioner of the Minnesota ...